The past decade has seen remarkable progress in the development of gene therapies for a broad range of human diseases. The ready accessibility and immune privilege of the eye, and the subretinal space in particular, has positioned ophthalmology at the forefront of this research, with recent innovations in gene therapy for the potential treatment of inherited retinal diseases (IRDs),1 diabetic retinopathy (DR),2 age-related macular degeneration (AMD),3 retinoblastoma,4 uveitis,5 hereditary optic neuropathy,6 and a variety of anterior segment disorders including glaucoma, corneal diseases, and aniridia.7 These advances culminated in the 2017 landmark FDA approval of voretegene neparvovec-rzyl (Luxturna; Spark Therapeutics) for the treatment of RPE65 mutation associated retinal dystrophy (Leber congenital amaurosis [LCA] type 2) — the first gene therapy approved in the United States for any inherited human disease.8 As growing research in the field of gene therapy propels us into a new era of clinical practice, it is helpful to understand the basic concepts and methods underlying these exciting and novel therapeutics. This article will review the general therapeutic approaches to gene therapy, common vectors employed to carry the transgene into the host cell, and basic techniques for delivery to the target tissue.
OVERVIEW
Broadly speaking, gene therapy involves the manipulation of cellular gene expression to prevent, halt, or reverse a disease process by introducing synthetic genetic material to the intracellular space.9 This is accomplished by first incorporating the genetic material — the transgene — into a delivery vector that will carry it into the host cell in a process called transduction (for viral vectors) or transfection (for nonviral vectors). The vector is designed to facilitate the transgene’s expression by placing it under the control of a tissue-specific promoter that will drive its expression in the target tissue — either constitutively or when activated by disease states (eg, hypoxia). In some cases, the vector may directly integrate the transgene into the host cell genetic code, allowing long-term expression and lasting therapeutic effects. In the treatment of retinal disease, this vector may be introduced to the target tissue by subretinal, intravitreal, or suprachoroidal injection.
THERAPEUTIC STRATEGIES
Basic strategies employed in gene therapy include:
- Gene augmentation/replacement: adding genetic material in cells of the target tissue to restore physiologic function;
- Gene silencing: preventing the production of dysfunctional protein from a pathologic gene;
- Translational read-through: restoring physiologic protein translation;
- Genome editing: revising a segment of the genetic code; and
- Selective toxicity: introducing “suicide” genes into pathologic cells to promote apoptosis.
Gene augmentation, the most widely implemented approach to gene therapy, underlies Luxturna’s mechanism of action. The strategy involves introducing genetic material to promote expression of physiologic or therapeutic protein product. Recessive, monogenic disorders are particularly amenable to this approach. Because these disorders are characterized by the absence of sufficient functional protein due to a single abnormal gene, the disease phenotype can be reversed by supplying a normal copy of the gene. This type of gene augmentation has been used in the development of investigational therapeutics for several IRDs, including autosomal recessive retinitis pigmentosa, Usher syndrome, LCA, Stargardt disease, achromatopsia, X-linked retinoschisis, bestrophinopathies, and choroideremia.1
Gene augmentation can additionally be employed in a gene-independent, protein-based fashion for multigenic or even nongenetic disorders. In this case, rather than restoring native protein expression, the transgene is instead designed to express non-native proteins that disrupt pathologic processes or recreate functionality, effectively transforming host cells into therapeutic “biofactories.” For example, transgenes designed to induce the retinal tissue to produce aflibercept-like or ranibizumab-like anti-VEGF proteins are currently being studied in the treatment of neovascular AMD and DR; a transgene expressing antitumor necrosis factor-alpha protein for the treatment of noninfectious uveitis is also under investigation. Optogenetic therapy for degenerative retinal diseases employs a similar approach to render ganglion or bipolar cells sensitive to light by the production of non-native opsins, effectively transforming them into artificial photoreceptors and restoring visual perception.10 These therapies have demonstrated encouraging results in recent clinical trials.
While gene augmentation is a promising strategy in the treatment of autosomal recessive diseases arising from the lack of functional protein expression, autosomal dominant diseases — such as autosomal dominant retinitis pigmentosa — typically involve dominant-negative pathologic protein expression that must be suppressed. Here, dysfunctional or toxic proteins expressed by gain-of-function mutations in one gene copy interfere with physiologic processes and cause disease pathogenesis, even in the context of a normal second copy of the gene. In such cases, pathologic gene expression may be suppressed by gene-silencing strategies. RNA interference (RNAi) describes a set of such techniques,11 whereby synthetic, noncoding RNA fragments (eg, microRNA, small interfering RNA, or short hairpin RNA) are designed to target and destroy messenger RNA (mRNA) transcribed from the abnormal gene, thereby preventing the translation of pathologic protein (Figure 1).
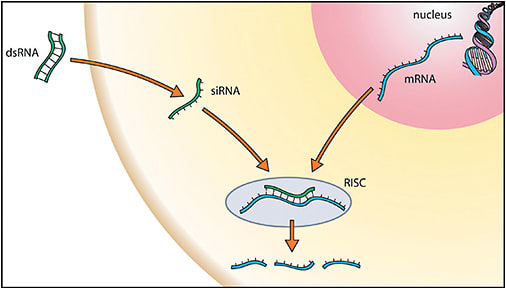
Antisense oligonucleotides are synthetic, single-strand RNA or DNA oligonucleotides that may accomplish a similar result by binding to mRNA and either degrading it or preventing its translation. Strategies of gene inactivation can be combined with gene augmentation by using the same plasmid to simultaneously introduce a replacement gene for physiologic protein expression alongside silencing of the pathologic protein expression (Figure 2).
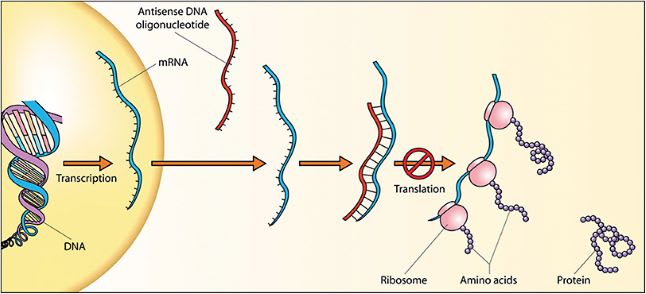
Aberrant post-transcriptional RNA splicing may result in nonsense mutations and pathologic stop codons that prematurely terminate mRNA translation and result in production of truncated, dysfunctional protein. Translational read-through strategies can address these problems, mainly in the form of translational read-through inducing drugs (TRIDs). These drugs work by increasing the ribosomal read-through activity of specific mRNA strands containing nonsense mutations, forcing the translation of full-length protein. Antisense oligonucleotides have also been employed to correct aberrant mRNA splicing and restore translation of functional protein.
Genome editing directly modifies the genome by precisely altering a target cell’s DNA code. The CRISPR/Cas editing system accomplishes this by creating double-stranded breaks in the target DNA and subsequently modifying the genetic code during the DNA repair process.12 This involves either introducing insertion/deletion mutation via nonhomologous end joining (thereby silencing gene expression), or the precise copying of new genetic code from a codelivered synthetic DNA template using error-free homologous recombination to correct pathologic mutations (restoring physiologic protein production). Genome editing may additionally be employed in a gene-independent fashion to reprogram cells into disease-resistant states to prevent disease onset or progression.13
In diseases resulting from abnormal cell proliferation (eg, retinoblastoma), selective toxicity may be employed to destroy pathologic cell populations while sparing healthy tissue. The strategy involves transducing target cells with genes that render target tissues sensitive to drug toxicity or immune-mediated destruction. For example, gene therapy utilizing the herpes simplex virus type 1 thymidine kinase transgene has been studied as a therapy for retinoblastoma by catalyzing the conversion of nontoxic prodrug ganciclovir into toxic triphosphate ganciclovir within the replicating cells, triggering apoptosis without damaging the surrounding tissue.14
DELIVERY VEHICLES
Once a particular approach to therapy has been chosen, an appropriate vehicle must be selected to deliver the transgenic material to the tissue in question. Viral vectors have classically been the method of choice, owing to their innate ability to selectively target and invade host cells, commandeer cellular machinery to translate their genome, and in some cases integrate their genetic material into the host genome. This approach may risk triggering immunologic reactions (known as gene therapy–associated uveitis) that could harm the local retinal tissue and limit therapeutic efficacy by degrading the viral vector. However, the immune-privileged status of the subretinal space mitigates such effects and minimizes clinically significant inflammatory response. Other spaces within the eye, such as the intravitreal space and suprachoroidal space, may also provide some degree of immune tolerance, particular for viral vectors that have been optimized for these routes of delivery.15
Lentiviruses and adeno-associated viruses (AAV) are currently the most widely studied viral vectors and have a strong track record of safety. Lentiviral vectors are derived from RNA retroviruses such as the human immunodeficiency virus 1 or equine infectious anemia virus. Lentiviral vectors can integrate into the host genome and have the capacity to carry large transgenes of up to 10 kb.16 On the other hand, AAV is a small single-stranded DNA virus of the parvovirus family. Adeno-associated virus vectors are nonreplicating, nonintegrating, and have relatively low immunogenicity.17 These features provide AAV vectors a superior safety profile over lentiviral vectors. Additionally, more than 100 AAV serotypes have been described, each with tropism for specific cell types, offering the advantage of more selective tissue targeting (Figures 3 and 4). However, AAV vectors are limited by their small carrying capacity of around 4.7 kb,17 requiring creative strategies to carry larger transgenes.18 Additionally, the widespread prevalence of prior virus exposure may somewhat increase risk of humoral immune response due to immune sensitization, though not prohibitively so.19
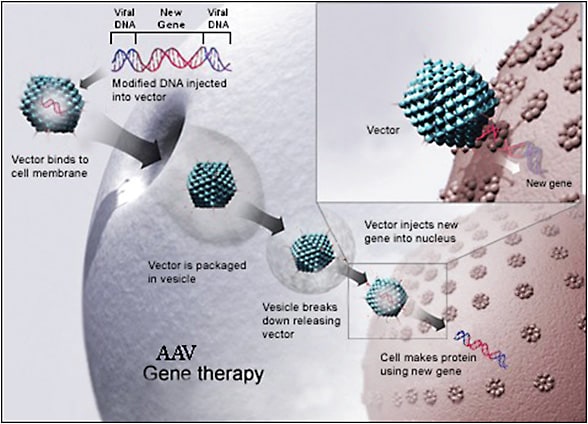
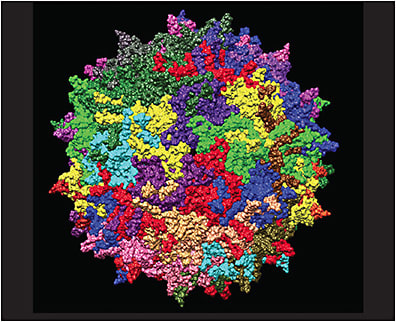
Nonviral vectors have also been investigated, including naked DNA, oligonucleotide, and RNA plasmids. Such vectors are less immunogenic and have larger carrying capacity. However, naked plasmids alone do not effectively transfect target cells.20 Electroporation may increase the efficacy of this approach by applying an electric field to the target cell and promoting transfection.21 Lipid and DNA nanoparticles are also being investigated as potential nonviral vectors for the transfection of large transgenes.22
ADMINISTRATION
Once a therapeutic strategy and appropriate delivery vector have been selected, the final step is to choose a method of administration. The most common techniques employed in the treatment of vitreoretinal disease include subretinal injection, intravitreal injection, and suprachoroidal injection (Figure 5). Subretinal injection is a surgical procedure requiring pars plana vitrectomy (PPV) with subsequent retinotomy and injection of the viral vector into the subretinal space.23 This approach allows delivery of the transgene directly to the target tissues and has been proven safe and efficacious — earning FDA approval for the administration of Luxturna. However, the procedure is highly invasive and involves subjecting the diseased retinal tissue to further trauma, including the necessary induction of a transient local retinal detachment. The procedure additionally carries the routine risks of pars plana vitrectomy, including cataract formation. An alternative technique without PPV has been described whereby a flexible cannula introduced into the suprachoroidal space through the pars plana is used to perform the subretinal injection using a small-gauge needle from the suprachoroidal side (Orbit Subretinal Delivery System; Gyroscope Therapeutics).
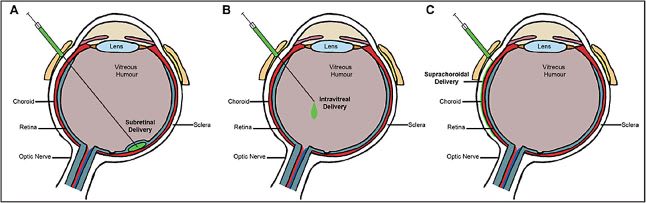
Intravitreal injections have also been investigated as a less invasive and more repeatable technique. Intravitreal injection holds particular promise in the delivery of gene therapy for diseases of the optic nerve such as Leber hereditary optic neuropathy.24 However, dilution in the vitreous cavity and the barrier effect of the inner limiting membrane limits the efficacy of this approach in the treatment of retinal disease.25 There is also evidence that intravitreal vector delivery may be more immunogenic, since the vitreous is not as immune-privileged as the subretinal space.26 However, a number of techniques may be implemented to mitigate these concerns, including optimized viral vectors via directed evolution or rational design, and the intravitreal approach has found growing success in clinical trials for certain retinal diseases.25
Finally, suprachoroidal techniques are currently under investigation.27 The technique of drug delivery though the suprachoroidal space has recently been corroborated through FDA approval of triamcinolone acetonide injectable suspension for suprachoroidal use (Xipere; Clearside Biomedical and Bausch + Lomb). With respect to gene therapy, suprachoroidal delivery offers the benefits of in-office administration and avoiding PPV. Clinical trials involving suprachoroidal administration of gene therapy are under way to evaluate the safety and efficacy of this approach.
MOVING FORWARD
The many triumphs of gene therapy in the past decade have opened the door to new avenues for treating blinding retinal disease. The number of gene therapies under investigation is increasing on a regular basis, and preliminary results from clinical trials currently under way have been largely encouraging. Exciting developments are on the horizon. The future of gene therapy is remarkably bright, with the potential to impact the lives of thousands as new strategies for restoring vision and preventing blindness are developed. RP
REFERENCES
- Shughoury A, Ciulla TA, Bakall B, Pennesi ME, Kiss S, Cunningham ET Jr. Genes and gene therapy in inherited retinal disease. Int Ophthalmol Clin. 2021;61(4):3-45. doi:10.1097/IIO.0000000000000377
- Wang JH, Roberts GE, Liu GS. Updates on gene therapy for diabetic retinopathy. Curr Diab Rep. 2020;20(7):22. doi:10.1007/s11892-020-01308-w
- Khanani AM, Thomas MJ, Aziz AA, et al. Review of gene therapies for age-related macular degeneration. Eye (Lond). 2022;36(2):303-311. doi:10.1038/s41433-021-01842-1
- Mandal M, Banerjee I, Mandal M. Nanoparticle-mediated gene therapy as a novel strategy for the treatment of retinoblastoma. Colloids Surf B Biointerfaces. 2022;220:112899. doi:10.1016/j.colsurfb.2022.112899
- Buggage RR, Bordet T. Gene therapy for uveitis. Int Ophthalmol Clin. 2021;61(4):249-270. doi:10.1097/IIO.0000000000000369
- Sahel JA, Newman NJ, Yu-Wai-Man P, et al. Gene therapies for the treatment of Leber hereditary optic neuropathy. Int Ophthalmol Clin. 2021;61(4):195-208. doi:10.1097/IIO.0000000000000364
- Amador C, Shah R, Ghiam S, Kramerov AA, Ljubimov AV. Gene therapy in the anterior eye segment. Curr Gene Ther. 2022;22(2):104-131. doi:10.2174/1566523221666210423084233
- Spark Therapeutics. FDA approves Spark Therapeutics’ Luxturna (voretigene neparvovec-rzyl), a 1-time gene therapy for patients with confirmed biallelic RPE65 mutation-associated retinal dystrophy. News release. December 19, 2017. Accessed February 6, 2023. https://sparktx.com/press_releases/fda-approves-spark-therapeutics-luxturna-voretigene-neparvovec-rzyl-a-one-time-gene-therapy-for-patients-with-confirmed-biallelic-rpe65-mutation-associated-retinal-dystrophy/
- Ciulla T, Pennesi ME, Kiss S, Cunningham ET Jr. DNA- and RNA-based gene therapies in ophthalmology. Int Ophthalmol Clin. 2021;61(3):3-16. doi:10.1097/IIO.0000000000000359
- Busskamp V, Picaud S, Sahel JA, Roska B. Optogenetic therapy for retinitis pigmentosa. Gene Ther. 2012;19(2):169-175. doi:10.1038/gt.2011.155
- Gemayel MC, Bhatwadekar AD, Ciulla T. RNA therapeutics for retinal diseases. Expert Opin Biol Ther. 2021;21(5):603-613. doi:10.1080/14712598.2021.1856365
- Burnight ER, Giacalone JC, Cooke JA, et al. CRISPR-Cas9 genome engineering: treating inherited retinal degeneration. Prog Retin Eye Res. 2018;65:28-49. doi:10.1016/j.preteyeres.2018.03.003
- Zhu J, Ming C, Fu X, et al. Gene and mutation independent therapy via CRISPR-Cas9 mediated cellular reprogramming in rod photoreceptors. Cell Res. 2017;27(6):830-833. doi:10.1038/cr.2017.57
- Hurwitz MY, Marcus KT, Chévez-Barrios P, Louie K, Aguilar-Cordova E, Hurwitz RL. Suicide gene therapy for treatment of retinoblastoma in a murine model. Hum Gene Ther. 1999;10(3):441-448. doi:10.1089/10430349950018887
- Bucher K, Rodríguez-Bocanegra E, Dauletbekov D, Fischer MD. Immune responses to retinal gene therapy using adeno-associated viral vectors — implications for treatment success and safety. Prog Retin Eye Res. 2021;83:100915. doi:10.1016/j.preteyeres.2020.100915
- Kumar M, Keller B, Makalou N, Sutton RE. Systematic determination of the packaging limit of lentiviral vectors. Hum Gene Ther. 2001;12(15):1893-1905. doi:10.1089/104303401753153947
- Daya S, Berns KI. Gene therapy using adeno-associated virus vectors. Clin Microbiol Rev. 2008;21(4):583-93. doi:10.1128/CMR.00008-08
- Trapani I. Adeno-associated viral vectors as a tool for large gene delivery to the retina. Genes (Basel). 2019;10(4):287. doi:10.3390/genes10040287
- Hareendran S, Balakrishnan B, Sen D, Kumar S, Srivastava A, Jayandharan GR. Adeno-associated virus (AAV) vectors in gene therapy: immune challenges and strategies to circumvent them. Rev Med Virol. 2013;23(6):399-413. doi:10.1002/rmv.1762
- Bordet T, Behar-Cohen F. Ocular gene therapies in clinical practice: viral vectors and nonviral alternatives. Drug Discov Today. 2019;24(8):1685-1693. doi:10.1016/j.drudis.2019.05.038
- Gehl J. Electroporation: theory and methods, perspectives for drug delivery, gene therapy and research. Acta Physiol Scand. 2003;177(4):437-447. doi:10.1046/j.1365-201X.2003.01093.x
- Conley SM, Naash MI. Nanoparticles for retinal gene therapy. Prog Retin Eye Res. 2010;29(5):376-397. doi:10.1016/j.preteyeres.2010.04.004
- Garafalo AV, Cideciyan AV, Héon E, et al. Progress in treating inherited retinal diseases: early subretinal gene therapy clinical trials and candidates for future initiatives. Prog Retin Eye Res. 2020;77:100827. doi:10.1016/j.preteyeres.2019.100827
- Yu-Wai-Man P, Newman NJ, Carelli V, et al. Bilateral visual improvement with unilateral gene therapy injection for Leber hereditary optic neuropathy. Sci Transl Med. 2020;12(573):eaaz7423. doi:10.1126/scitranslmed.aaz7423
- Ross M, Ofri R. The future of retinal gene therapy: evolving from subretinal to intravitreal vector delivery. Neural Regen Res. 2021;16(9):1751-1759. doi:10.4103/1673-5374.306063
- Li Q, Miller R, Han PY, et al. Intraocular route of AAV2 vector administration defines humoral immune response and therapeutic potential. Mol Vis. 2008;14:1760-1769.
- Kansara V, Muya L, Wan CR, Ciulla TA. Suprachoroidal delivery of viral and nonviral gene therapy for retinal diseases. J Ocul Pharmacol Ther. 2020;36(6):384-392. doi:10.1089/jop.2019.0126