After a 25-year preclinical journey, regulatory agency approval in the United States and in Europe of voretigene neparvovec-rzyl (Luxturna; Spark Therapeutics) marked the transition of ocular gene therapy from the bench to the bedside.1 The success of Luxturna in RPE65-associated inherited retinal degeneration (IRD) gives confidence that gene therapy may serve as a drug delivery platform for the long-term treatment of noninherited ocular disorders beyond IRDs. For example, in more common disorders, such as exudative or wet age-related macular degeneration (AMD), diabetic retinopathy (DR), and diabetic macular edema (DME), a gene-therapy platform may be utilized to deliver an anti-vascular endothelial growth factor (anti-VEGF) molecule to obviate the need for repeated intravitreal (IVT) injections.2 An understanding of the underlying concepts of gene therapy will allow continued development of this platform for a wide range of ocular diseases.
Broadly speaking, gene therapy for ocular disorders requires consideration of 4 interrelated components: (1) the vector, (2) the route of delivery, (3) the specific transgene, and (4) manufacturing.1 In the early 1990s, replication-defective retroviruses were used as the earliest gene-delivery vectors. However, genotoxicity from and inflammatory response to retrovirus administration promptly restricted their clinical practicality. A decade later, adeno-associated virus (AAV) vectors derived from nonpathogenic replication-deficient parvovirus emerged as a safe and effective tool for gene delivery. The current upsurge of clinical gene therapy is based primarily on this AAV technology. The differences among various serotypes of novel AAV vectors enables improved efficiency in targeting a broad range of retinal disorders via the IVT, subretinal (SR), and suprachoroidal (SC) routes (Table 1).2
VECTOR | FEATURES | ADVANTAGES | LIMITATIONS |
---|---|---|---|
Nonviral Gene Delivery |
|
|
|
Lentiviral Vectors (LV) |
|
|
|
Adenoviral Vectors (Ad) |
|
|
|
Adeno-Associated Viral Vectors (AAV) |
|
|
|
Second-Generation AAV |
|
|
|
ROUTES OF DELIVERY
The route of delivery and vector considerations are profoundly interwoven with ocular gene therapy (Figure 1).1 Theoretically, ocular gene delivery may be accomplished via various routes, including topical drops, periocular routes (eg, sub-Tenon or subconjunctival injection), intracameral injection, IVT injection, SC injection, or SR delivery, the last of which requires surgery. In practice, the particular disease (eg, IRD or wet AMD), the exact target cell (eg, RPE, rod, or cone cell) and the vector delivery system (eg, AAV8 or AAV7m8) dictate the route of administration. Intravitreal injection and SR surgical delivery are the 2 most common routes of administration for viral-based gene therapy for retinal diseases. Suprachoroidal delivery is also emerging as a potential in-office gene therapy platform, although clinical experience has been limited.
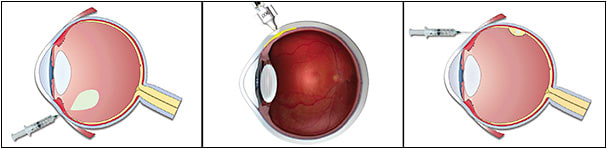
Intravitreal Injection
Intravitreal injection is an established and quite safe route for pharmacotherapy administration to the eye. Here, the vector is injected directly into the vitreous, typically in an office-based setting. Because IVT injection of viral vectors transduces predominantly retinal ganglion cells (RGCs), it is the preferred route for targeting the inner retina. Although IVT delivery appears to be a direct path to the retina, there are anatomic barriers that prevent diffusion of viruses into the retina, most especially the inner limiting membrane (ILM) found in primate eyes.3 For example, the lack of clinically successful therapies using IVT AAV2 gene therapy is most likely related, at least in part, to the ILM barrier to efficient AAV2 retinal transduction. In damaged or diseased retinas, such as in wet AMD, there may be a potentially increased efficiency of retinal transduction following IVT injection due to an increased passage of the vectors from the vitreous toward the photoreceptors. Strategies to enhance transduction of AAV following IVT administration include mild enzymatic digestion or surgical peeling of the ILM.4 Importantly, second-generation and third-generation novel AAV vectors are being designed to overcome these anatomical barriers to retinal transduction following IVT delivery.5
Subretinal Delivery
With SR injection, the vector is delivered in a localized SR bleb between the retinal pigment epithelium (RPE) and the photoreceptor layer in a surgical procedure carried out in an operating room.6 This can be undertaken either following a pars plana vitrectomy via a transretinal route, or, alternatively, a SR bleb can be made from the external SC space using innovative surgical devices.7 Subretinal injection of vectors transduces predominantly RPE cells, photoreceptors, and, to a lesser extent, Müller cells. Compared to IVT injection, SR delivery is more invasive and potentially has more complications; however, SR administration provides the most direct access to photoreceptors and the RPE. Although the spread of virus beyond the bleb formed at the injection site is limited, for most cases this is adequate for the delivery of the therapeutic transgene.
Suprachoroidal Injection
Suprachoroidal administration involves delivery of a vector into a potential space between the sclera and the choroid. It offers a less invasive alternative to SR injections and overcomes the ILM barrier associated with IVT injection.8 Although experience with this route of administration is limited, animal studies have demonstrated the potential safety and efficacy of SC delivery of vectors to the retina, especially to the outer retinal cells.
VECTOR CONSIDERATIONS
Over the past 4 decades, a variety of vector systems have been employed for gene therapy,1 including both nonviral strategies like liposomes and nanoparticles and modified viral vectors, most notably lentivirus, adenovirus, and adeno-associated viruses.
Nonviral Approaches
The most widely employed nonviral strategies include lipid-based and polymer-based carriers.9,10 These approaches offer several advantages over viral vectors, such as a theoretically more favorable safety profile due to a lower risk for immunogenicity or insertional mutagenesis associated with viral vectors, the ability to deliver much larger transgenes, and the potential for repeated and greater dosing. The challenge for all nonviral delivery systems remains the need to overcome the physical barriers to transfection. Moreover, as nonviral strategies are not self-replicating systems, this approach will likely require repeated administrations for long-term durability and as such, are typical not considered a “one-and-done” administration.11
Liposomes
Liposomes comprise amphiphilic molecules, such as phospholipids and cholesterol.11 Inclusion of positively charged or titratable components can promote complexation with negatively charged DNA. Because cellular membranes are composed of a phospholipid bilayer, liposomes can be designed to fuse with and overcome the cell membrane barrier. Indeed, liposomes can mediate uptake into RPE cells following SR injection.
Solid lipid nanoparticles
Solid lipid nanoparticles are composed of an aqueous dispersion of a layer of surfactants surrounding a solid lipid core, and they range in size from 50 nm to 1,000 nm.12 Subretinal injections of solid lipid nanoparticles have resulted in transfection of RPE cells.
Bioerodible polymers
Bioerodible polymers, such as polyethyleneimine; polyesters like poly(lactic acid) (PLA) and poly(lactic-co-glycolic acid) (PLGA); chitosan; and hyaluronic acid have been investigated for ocular gene therapy. However, polyethyleneimine-based polymers have not shown efficient delivery to the retina following IVT injection and as such have gone out of favor.
Viral Approaches
Lentiviruses
Lentiviruses (LV) are single-stranded RNA viral vectors that can transduce both dividing and nondividing cells.13 Because most LVs integrate their DNA into the chromosome of target cells, they can mediate sustained transgene expression, theoretically forever, following a single administration, even in dividing cells. However, with integration into the host genome, insertional mutagenesis remains a concern with LVs. Several approaches are being developed to address this liability, including, for example, inactivation of the 3′ long terminal repeat to generate self-inactivating vectors.13 Lentivirus gene therapy approaches can overcome a major constraint of the limited packaging capacity of AAV vectors. Lentiviruses can accommodate large transgenes up to 10 kilobases (kb) (eg, ABCA4 gene), whereas AAVs have a limit of less than 5 kb. Lentiviruses can effectively transduce RPE cells and, to a much lesser extent, photoreceptors and other retinal cells.
Adenovirus
Adenoviruses (Ad) are double-stranded DNA vectors that can proficiently transduce both dividing and nondividing cells.14 Ad’s have the largest payload capacity of any currently used viral vector systems, with the ability to package genetic material of up to 37 kb. Unlike LVs, Ad genetic material is nonintegrating and remains episomal, reducing substantially the risk for insertional mutagenesis. However, because the payload does not integrate into the target cell genome, Ad gene therapy is most useful for transduction of and long-term transgene expression in postmitotic cells. In actively dividing cells, the Ad episomes carrying the genetic material are diluted with each cell division and the transgene product production is markedly reduced.
There are more than 50 adenovirus serotypes with a wide range of cellular tropisms. The most commonly used serotypes — Ad5 and Ad2 — can transduce RPE and, to a lesser extent, photoreceptors. The Ad5 serotype serves as the basis for many current Ad-based clinical gene therapy programs. For example, a phase I clinical trial with Ad5 injected intravitreally to produce pigment epithelium derived factor in patients with wet AMD established a reasonably safe side-effect profile and some evidence of sustained therapeutic effect.15
The major drawback of Ad gene therapy is the considerable immune response to the vector that can substantially limit transgene expression, even when delivered via SR injection.16 For example, cytotoxic T-cells have been shown to remove transduced cells expressing any Ad proteins. Novel helper-dependent Ad vectors containing only the inverted terminal repeats and the packaging recognition signal, along with the transgene, have been developed to overcome this host immune response and allow for long-term transgene expression.17
Adeno-associated virus vectors
Adeno-associated virus (AAV) vectors have emerged as the predominant vector for ocular gene therapy.18 Adeno-associated virus is a small (19 nm to 21 nm), nonenveloped, DNA virus belonging to the Parvoviridae family. AAV requires a helper virus for replication and is thus considered to be nonpathogenic. Adeno-associated viruses can transduce both dividing and nondividing cells and have favorable retinal cell transduction properties. Similar to Ad vectors, the genetic material following AAV transduction remains episomal, reducing the potential for mutagenesis from genomic integration (a risk associated with LV).
Among the viral vectors, AAV has the most limited packaging capacity of around 4.8 kb.18 Moreover, the numerous regulatory elements associated with AAV gene expression further reduce the capacity of the transgene of interest to around 3 kb. Some of the cDNAs of the genes that are responsible for IRDs are much larger than this limit and thus are not suited to current AAV gene therapy approaches. For example, the ABCA4 and MYO7A are each roughly 7 kb in length, making them impossible to insert into AAV vectors.
Adeno-associated virus genome is packaged within an icosahedral capsid containing 3 open reading frames (ORFs) bordered by inverted terminal repeats (ITRs). The rep ORF encodes for proteins (Rep40, Rep52, Rep68, and Rep78) that are essential for viral replication, transcriptional regulation, genome integration, and virion assembly. The cap ORF encodes 3 structural proteins — VP1, VP2, and VP3 — which comprise the 60-subunit AAV icosahedral capsid. The 3 capsid proteins contain a common β-barrel domain but different N terminal extensions. Variable loops within the capsid proteins create specific surface topologies for each AAV serotype; these loops facilitate the interactions of the viral particle with the host. The AAV variants differ in their capsid components and thus exhibit distinct cellular tropism, transduction efficiency, and immunogenicity.
About a dozen different naturally occurring AAV serotypes have been identified in primates with more than 100 variants.18 Naturally occurring AAV1, AAV2, AAV4, AAV5, AAV6, AAV7, AAV8, and AAV9 all display tropism for retinal tissue. All of these serotypes efficiently transduce RPE, especially following SR delivery; however, transduction of photoreceptors and other cell types within the retina differs among the various serotypes. The naturally occurring serotypes AAV2, AAV5, and AAV8 have been most widely studied for use as ocular gene-therapy vectors. These 3 vectors work most efficiently via a SR delivery route, because transduction of retinal cells following IVT injection is limited by the ILM as well as pre-existing neutralizing antibodies. For example, depending on geographic region, 30% to 60% of humans have neutralizing antibodies (nAbs) to AAV2;19 these nAbs have the potential to severely limit cellular transduction and transgene protein production.
Interestingly, capsid proteins can be exchanged among various AAV serotypes. Transient transduction of the different vector components allows for exchange of capsids between different serotypes resulting in hybrid or “pseudotyped” AAV vectors. For example, many recombinant vectors in use today are composed of components of the AAV2 serotype combined with the capsids of AAV1 (AAV2/1), AAV4 (AAV2/4), AAV5 (AAV2/5), AAV6 (AAV2/6), AAV7 (AAV2/7), AAV8 (AAV2/8), and AAV9 (AAV2/9). In addition to attaining the desired cellular tropism and enhancing transduction efficiency, pseudotyping can also circumvent pre-existing immunity to certain AAV serotypes that could affect the transduction efficacy and potentially the safety of a ocular gene therapy.
All AAV pseudotypes can transduce RPE, with AAV2/1, AAV2/4, and AAV2/6 being the most specific. In contrast, only AAV2/5, AAV2/7, AAV2/8, and AAV2/9 efficiently transduce photoreceptors, with AAV2/8 and AAV2/9 being most efficient across a number of species. The pseudotypes AAV2/5, AAV2/8, and AAV2/9 transduce the highest percentage of cone photoreceptors.20
Recombinant AAV (rAAV) vectors have been designed for gene therapy by replacing the rep and cap genes with the transgene cassette of interest18 (eg, RPE65 in the case of Luxturna, and anti-VEGF protein in the case of RGX-314 [REGENXBIO] and ADVM-022 [Adverum Biotechnologies]). The rep and cap ORFs are then provided during the AAV manufacturing process via helper plasmids. rAAV2, the first serotype to be successfully used for gene therapy, efficiently transduces RPE cells following SR delivery (eg, Luxturna).
Beyond naturally occurring AAVs: rational design and directed evolution
Recently, the range of AAV vectors available for ocular gene therapy has been expanded beyond naturally occurring serotypes to second-generation vectors. These new vectors have been designed or isolated explicitly to have greater transduction efficiency, altered tropism, and/or improved immunogenicity profile when compared to their naturally occurring counterparts.1 Two different approaches have been used to generate these novel vectors: rational design and directed evolution.
Rational design leverages knowledge of structure and function relationships to modify the virus capsid structure directly for the desired outcome. Examples of rational design include the introduction of capsid mutations to reduce viral degradation, to eliminate antibody binding epitopes, or to direct vector tropism for specific cell types. Rational design has been used to generate variants, by site-directed mutagenesis, of surface-exposed tyrosine residues on AAV2 to prevent capsid phosphorylation and subsequent ubiquitination and proteasome-mediated degradation; this results in enhanced nuclear transport and increased transgene expression following target cell transduction.21 The vectors AAV2, AAV8, and AAV9 carrying these mutations have been shown to have increased transduction efficiency both in vitro and in vivo.
In contrast to rational design, the directed evolution approach to novel vector design assumes no prior knowledge of structure and function relationships; rather, directed evolution relies on the introduction of random genetic variability on a grand scale, used in conjunction with selection pressure, to accumulate mutations that result in the desired improvement in the vector.22 Directed evolution is a process that, in effect, mimics natural selection, only on a much grander scale and at a considerably accelerated rate. Large AAV libraries are generated by random mutagenesis of the capsid proteins and then screened in vivo for transduction efficiency or specificity (eg, screening for vectors that efficiently transduce retinal cells following IVT injection). This approach has been used to generate novel AAV vectors that can more effectively cross biological barriers, such as the ILM, and target specific cell types.
An example of a novel vector developed via directed evolution that is showing great promise in wet AMD patients is AAV.7m8. The AAV.7m8 vector is a unique AAV2 capsid variant that is capable of efficient and robust gene delivery to all retina layers in both mice and primates following a single IVT injection.22 With the ILM serving as a robust barrier to viral transduction, naturally occurring AAV2 requires a complex surgical SR delivery approach for use in ocular gene therapy. The AAV.7m8 vector overcomes this limitation because it was explicitly designed, through a process of in vivo directed evolution, to enhance retinal penetration and transduction from the vitreous, in an effort to optimize IVT AAV delivery.
Promoters and enhancers for viral vectors
In addition to exploiting vector variants, another strategy for cell-specificity is the use of cell-type–specific promoters and enhancers. Following viral vector transduction and insertion of genetic material into the target cell, specific promotors allow for the transcription and production of the desired transgene only in selected cells. Most gene therapy approaches use strong ubiquitous promoters such as the cytomegalovirus (CMV) or the chimeric chicken β-actin CMV enhancer and promoter. With these promotors, all target cells that are transduced by the viral vector will theoretically produce the transgene protein. For example, ubiquitous promotors would be most appropriate for anti-VEGF gene therapy. In certain instances, however, it may be appropriate to limit the expression of the gene to a specific target cell type (eg, RPE, rods, or cones) to prevent unwanted side effects from the transgene production. Most gene therapies for IRDs, for example, are intended to target defects in very specific cells (eg, photoreceptors or the RPE). The rhodopsin kinase 1 and interphotoreceptor retinoid binding protein promoters can drive expression of transgenes in both cone and rod photoreceptors (and not in RPE or ganglion cells). For rod-specific expression, the rhodopsin promoter can be employed; cone-specific expression can be achieved using cone arrestin, blue opsin, or red/green opsin promoters.23 Similarly, RPE-specific expression can be driven by the RPE65 promoter, and specific expression can be achieved in ON bipolar cells and Müller cells using cell-type–specific promoters.24 With these cell-specific promotors, only the cells that typically recognize those particular promotors will produce the transgene product. Although other cell types may be transduced by the viral vector, without recognition of the promotor sequence, the genetic material is not transcribed and the protein is not produced in those cells.
CONCLUSIONS
Taking gene therapy beyond IRDs into the realm of AMD, DR, and DME will require thoughtful consideration of the features of the gene therapy vector serotypes, an understanding of the transgene constructs (Figure 2) and the associated promotors and enhancers, as well as optimization of the route of delivery.1 Learnings from preclinical studies and from patients who have been treated with gene therapies have resulted in the development of novel vectors with enhanced tropisms for retinal cells using specific routes of delivery. Technologic advances have allowed more standardized and novel routes of ocular delivery of these vectors to maximize retinal cell transduction and transgene protein production. We stand at the horizon of a paradigm shift of exploiting gene therapy, not only to correct genetic abnormalities, but also as a drug delivery platform to provide a protein that is not usually produced by the eye to treat more common ocular disorders.1 RP

REFERENCES
- Hou AY, Kiss S. An update on ocular gene therapy for monogenic and multifactorial retinal diseases. J Vit Dis. 2019;3(5):366-377.
- Kiss S. Ocular gene therapy: focus on exudative age-related macular degeneration and other non-inherited disorders. Presented at the 123rd Annual Meeting of the American Academy of Ophthalmology; October 2019; San Francisco, CA.
- Dalkara D, Kolstad KD, Caporale N, et al. Inner limiting membrane barriers to AAV mediated retinal transduction from the vitreous. Mol Ther. 2009;17(12):2096-2102.
- Takahashi K, Igarashi T, Miyake K, et al. Improved intravitreal AAV-mediated inner retinal gene transduction after surgical internal limiting membrane peeling in cynomolgus monkeys. Mol Ther. 2017;25(1):296-302.
- Auricchio A, Kobinger G, Anand V, et al. Exchange of surface proteins impacts on viral vector cellular specificity and transduction characteristics: the retina as a model. Hum Mol Genet. 2001;10(26):3075-3081.
- Bruewer AR, Mowat FM, Bartoe JT, Boye SL, Hauswirth WW, Petersen-Jones SM. Evaluation of lateral spread of transgene expression following subretinal AAV-mediated gene delivery in dogs. PLoS One. 2013;8(4):e60218.
- Peden MC, Min J, Meyers C, et al. Ab-externo AAV-mediated gene delivery to the suprachoroidal space using a 250 micron flexible microcatheter. PLoS One. 2011;6(2):e17140.
- Ding K, Shen J, Hafiz Z, et al. AAV8-vectored suprachoroidal gene transfer produces widespread ocular transgene expression. J Clin Invest. 2019;130:4901-4911.
- Kiss S. A novel approach to ocular gene therapy: evaluation of suprachoroidally administered non-viral DNA nanoparticles. Presented at the 42nd Annual Meeting of the Macula Society; March 2019; Bonita Spring, FL.
- Ramamoorth M, Narvekar A. Non viral vectors in gene therapy - an overview. J Clin Diagn Res. 2015;9(1):GE01-GE06.
- Pitkanen L, Ruponen M, Nieminen J, Urtti A. Vitreous is a barrier in nonviral gene transfer by cationic lipids and polymers. Pharm Res. 2003;20(4):576-583.
- Bloquel C, Bourges JL, Touchard E, Berdugo M, BenEzra D, Behar-Cohen F. Non-viral ocular gene therapy: potential ocular therapeutic avenues. Adv Drug Deliv Rev. 2006;58(11):1224-1242.
- Yáñez-Muñoz RJ, Balaggan KS, MacNeil A, et al. Effective gene therapy with nonintegrating lentiviral vectors. Nat Med. 2006;12(3):348-353.
- Boye SE, Boye SL, Lewin AS, Hauswirth WW. A comprehensive review of retinal gene therapy. Mol Ther. 2013;21(3):509-519.
- Campochiaro PA, Nguyen QD, Shah SM, et al. Adenoviral vector-delivered pigment epithelium-derived factor for neovascular age-related macular degeneration: results of a phase I clinical trial. Hum Gene Ther. 2006;17(2):167-176.
- Reichel MB, Ali RR, Thrasher AJ, Hunt DM, Bhattacharya SS, Baker D. Immune responses limit adenovirally mediated gene expression in the adult mouse eye. Gene Ther. 1998;5(8):1038-1046.
- Parks RJ, Chen L, Anton M, Sankar U, Rudnicki MA, Graham FL. A helper-dependent adenovirus vector system: removal of helper virus by Cre-mediated excision of the viral packaging signal. Proc Natl Acad Sci U S A. 1996;93(24):13565-13570.
- Schön C, Biel M, Michalakis S. Retinal gene delivery by adeno associated virus (AAV) vectors: strategies and applications. Eur J Pharm Biopharm. 2015;95(Pt B):343-352.
- Calcedo R, Vandenberghe LH, Gao G, Lin J, Wilson JM. Worldwide epidemiology of neutralizing antibodies to adeno-associated viruses. J Infect Dis. 2009;199(3):381-390.
- Manfredi A, Marrocco E, Puppo A, et al. Combined rod and cone transduction by adeno-associated virus 2/8. Hum Gene Ther. 2013;24(12):982-992.
- Petrs-Silva H, Dinculescu A, Li Q, et al. High-efficiency transduction of the mouse retina by tyrosine-mutant AAV serotype vectors. Mol Ther. 2009;17(3):463-471.
- Dalkara D, Byrne LC, Klimczak RR, et al. In vivo–directed evolution of a new adeno-associated virus for therapeutic outer retinal gene delivery from the vitreous. Sci Transl Med. 2013;5(189):189ra176.
- Komáromy AM, Alexander JJ, Cooper AE, et al. Targeting gene expression to cones with human cone opsin promoters in recombinant AAV. Gene Ther. 2008;15(14):1049-1055.
- Macé E, Caplette R, Marre O, et al. Targeting channelrhodopsin-2 to ON-bipolar cells with vitreally administered AAV restores ON and OFF visual responses in blind mice. Mol Ther. 2015;23(1):7-16.
Editor’s note: This article is part of a special edition of Retinal Physician that was supported by REGENXBIO.