Although gene augmentation and gene editing have received much attention in the retina community recently, RNA therapy (targeting RNA downstream from the gene) has generated great interest in the broader medical community, after approvals for devastating systemic disorders such as spinal muscular atrophy (nusinersen/Spinraza; Biogen) and Duchenne muscular dystrophy (eteplirsen/Exondys 51 and golodirsen/Vynondys 53; Sarepta). However, the retina field has played a leading role in RNA therapy, with the first approval for an antisense oligonucleotide (ASO) in 1998 (fomivirsen/Vitravene; Isis Pharmaceuticals) for intravitreal treatment of cytomegalovirus (CMV) retinitis. The retina is a prime target for development of both gene therapies and RNA therapies, given the large number of monogenic disorders, accessibility to target cell delivery, and the noninvasive ability to monitor for disease progression or therapeutic response.
RNA therapy has the potential to very specifically modify faulty gene products by targeting their RNA precursors. Notably, RNA therapies differ from protein-targeting therapeutic oligonucleotides, such as pegaptanib sodium (Macugen; Bausch + Lomb) which binds the 165 isoform of vascular endothelial growth factor (VEGF)-A for the treatment of neovascular age related macular degeneration (nAMD). Also, unlike voretigene neparvovec-rzyl (Luxturna; Spark Therapeutics) and many investigational ocular gene augmentation therapies, most RNA therapies are mutation specific, administered intravitreally, have potential for panretinal effect, and require repeat administration.
NONCODING RNA: miRNA, siRNA, shRNA
In the cell nucleus, genes consist of both DNA exons, which encode proteins, and introns, which are not expressed but contain regulatory elements. DNA is transcribed by an RNA polymerase into pre-messenger RNA (pre-mRNA; Figure 1), from which the noncoding intronic regions are spliced out to form mRNA. This mRNA is then transported through the nuclear pore complex to cytoplasmic ribosomes to be translated into polypeptide chains. Noncoding RNAs (ncRNAs) are inherent in the genome and have essential regulatory functions. Disease states can lead to differential expression of these ncRNAs and, in turn, disease development and progression. As such, ncRNAs are prime targets for clinical application. The majority of RNA therapeutics currently in development are synthetic ncRNAs.
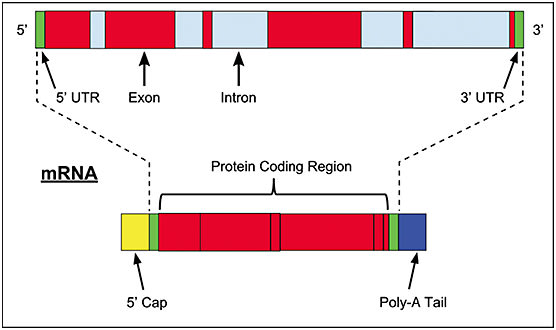
MicroRNA
Since their discovery, microRNA (miRNA), a class of ncRNAs which regulate post-translational gene expression, have been targeted as a treatment strategy. In the nucleus, an RNA polymerase transcribes DNA into pri-miRNA, which includes a double-stranded hairpin structure that is processed further into pre-miRNA by Drosha (a ribonuclease with double-stranded RNA [dsRNA] specificity).1 Pre-miRNA is then transported through the nuclear pore complex into the cytoplasm, where the loop of the hairpin is cleaved by Dicer (another dsRNA-specific ribonuclease).1 A double-stranded miRNA results, and one strand then interacts with the Argonaute protein to form an RNA-induced silencing complex (RISC).1 Through the miRNA “guide strand,” this enzymatic complex then recognizes and cleaves target mRNA, with repression of its translation.
In the retina, miRNAs have been implicated in complications of AMD, through regulation of angiogenesis, oxidative stress, immune response, and inflammation.2 Studies have shown alterations in miRNA activity levels involved with choroidal neovascularization development, as well as reduction of neovascularization with their regulation.3-5 With these findings, miRNA could serve as biomarkers of disease activity or as targets of therapeutic strategies. For example, synthesized miRNAs could guide the RISC complex to a therapeutic target RNA, inhibiting its translation. However, miRNAs imperfectly bind to their complementary target, requiring only a 2–8 nucleotide seed sequence to identify and inhibit translation of its target.1 Due to the nature of this binding, a single miRNA can potentially bind and target multiple mRNAs, enhancing their potential therapeutic capabilities but also increasing the risk of off-target effects.1
Small Interfering RNA
Similarly, RNA interference (RNAi) is a developed technique to silence proteins in a sequence-specific manner by inhibiting mRNA and consequently reducing protein expression. RNAi does not intrinsically occur in humans; however, the above miRNA pathway and RNAi share common proteins that can be activated through synthesized molecules to bind and cleave their targets.1 The main functional mediator for RNAi is small-interfering RNA (siRNA). These are short dsRNA oligonucleotides, from which the antisense strand becomes incorporated into RISC, in the cytoplasm of the cell. The activated RISC uses this antisense strand of the siRNA to guide it to the targeted complementary mRNA, ultimately leading to its degradation. This activated complex can continue to degrade additional mRNA targets until siRNA are diluted or degraded within the cell.1,6
Attempts have been made to use siRNA molecules to target and silence VEGF, VEGF receptor, and related pathway genes in animal models. However, clinical trials with siRNA investigational agents (bevasiranib, originally called Cand5, from OPKO Health/Acuity Pharmaceuticals; AGN211745, originally called Sirna-027, from Allergan; and PF-4523655 from Pfizer and Quark Pharmaceuticals) failed to reach primary endpoints compared with other anti-VEGF agents. More recently, Regeneron and Alnylam pharmaceuticals announced a collaboration to develop RNAi for ocular disease.
Short Hairpin RNAs
Another form of RNAi involves short hairpin RNAs (shRNA; Figure 2). ShRNAs have similar structure to miRNAs, and they are processed by the same pathway, generating siRNAs. While most RNA therapies are injected intravitreally and require repeat administration, shRNAs can be encoded into DNA to be introduced into the cell nucleus by a vector for continuous production. Following transcription, the shRNA would be exported to the cytoplasm to be processed by Dicer into siRNA duplexes. At this point, it would function similarly to the exogenously delivered siRNAs mentioned above, forming a RISC complex and binding to complementary mRNA for target-specific degradation.7,8
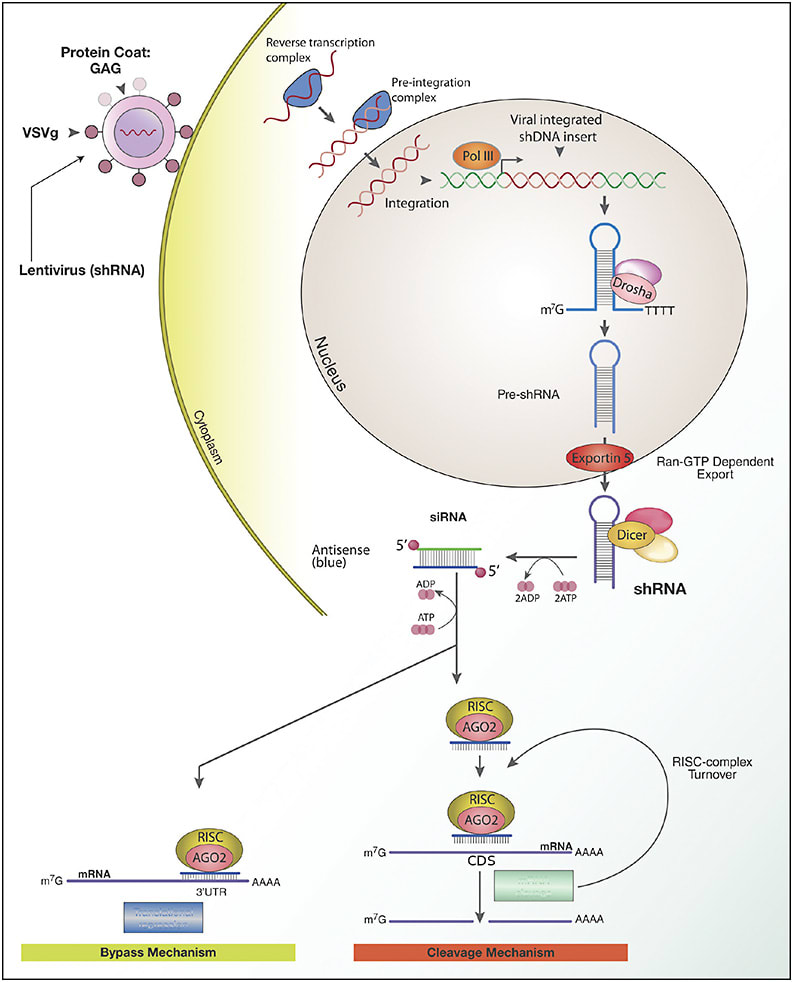
Use of shRNA is a promising strategy. One group developed an AAV2/8 vector to express shRNAs that target VEGF mRNA, demonstrating reduction of choroidal neovascularization in a mouse model.9 This strategy may be especially useful in cases of autosomal-dominant disorders. Gene therapy in many of these cases faces the obstacle of needing to suppress a mutation, causing a toxic gain of function. Investigation is under way for the most common autosomal dominant inherited retinal disease (IRD), autosomal-dominant retinitis pigmentosa (adRP), using shRNA in a “knockdown and replace” strategy, by which shRNA targeting the causative mutated form of the gene rhodopsin (RHO) for silencing is co-packaged with a resistant form of human RHO in a single vector. In canine models, this strategy was shown to provide complete and long-term protection for rods against an RHO mutation.10 Iveric bio is currently working to apply this model to humans using a mutation independent gene therapy, IC-100.11
CLINICAL TRIAL NUMBER | RNA THERAPEUTIC STRATEGY | DISEASE |
NCT00363714 | siRNA (SIRNA-027/AGN211745) | Subfoveal choroidal neovascular membranes secondary to age-related macular degeneration |
NCT00306904 | siRNA (Cand5/Bevasiranib) | Diabetic macular edema |
NCT01445899 | siRNA (PF-04523655) | Diabetic macular edema |
NCT01064505 | siRNA (QPI-1007) | Optic nerve atrophy in nonarteritic anterior ischemic optic neuropathy |
NCT02947867 | ASO (Aganirsen) | Ischemic central retinal vein occlusion to prevent neovascular glaucoma |
NCT03780257 | ASO (QR-421a) | Retinitis pigmentosa/Usher syndrome type 2 |
NCT04123626 | ASO (QR-1123) | Autosomal dominant retinitis pigmentosa |
NCT03815825 | ASO (IONIS-FB-LRx) | Geographic atrophy secondary to age-related macular degeneration |
NCT03913143 | ASO (Sepofarsen/QR-110) | Leber congenital amaurosis |
ANTISENSE OLIGONUCLEOCTIDES
Initially noted to be very effective in treatment of CMV retinitis, even in cases resistant to conventional therapies, fomivirsen was withdrawn from the market in 2006, with the advent of highly active antiretroviral therapy dramatically reducing the incidence of CMV retinitis.12 ASOs generally are single-stranded, synthetic RNAs (or DNAs) engineered to selectively bind to complementary target mRNA.1 Several postbinding mechanisms can then occur. Binding to RNA can lead to translation arrest (by binding near the initiation codon) without promoting RNA degradation, promotion of RNA degradation (through recruitment of RNase H), prevention of 5’ cap formation (which plays an important role in the ribosomal recognition of mRNA), or alteration of pre-mRNA splicing.13
For example, ASOs can restore ABCA4 splicing defects caused by deep intronic mutations associated with Stargardt macular dystrophy.14 Similarly, ProQR is developing an ASO that addresses a common intronic mutation that causes an aberrant splice site in ABCA4, which, in turn, leads to exclusion of exon 39.15 In addition, ProQR has demonstrated promising results in a phase 1/2 trial involving quarterly dosing of sepofarsen, an ASO that addresses the p.Cys998X mutation in the CEP290 gene responsible for Leber congenital amaurosis 10 (LCA10).
TRANSLATIONAL READ-THROUGH INDUCING DRUGS AND EUKARYOTIC RIBOSOMAL SELECTIVE GLYCOSIDES
Nonsense mutations leading to premature stop codons are not uncommon, and they halt functional protein production. With complete loss of functional protein, these phenotypes can be more severely affected than with other mutations in which some functional protein remains. Translational read-through inducing drugs (TRIDS) such as eukaryotic ribosomal selective glycosides (ERSG) increase ribosomal read-through activity of mRNA with these premature stop codon mutations, enabling production of full-length functional proteins.16 Eloxx pharmaceuticals is developing subcutaneously delivered small molecule ERSGs (which lack the typical toxicities of aminoglycosides) to address a variety of these premature stop codon mutations at different positions within a given gene. Consequently, ERSGs are not mutation-specific like other RNA therapeutics. Eloxx’s lead compound is ELX-02, which is currently undergoing phase 2 study in cystic fibrosis and cystinosis. In addition, Eloxx pharmaceuticals plans to apply this strategy to rare IRDs caused by nonsense mutations, with initial focus on Usher syndrome.16
CONCLUSION
RNA-based gene therapy is an exciting and rapidly evolving field with potential to effectively treat a wide range of retinal diseases. Many of these RNA-directed treatments are mutation specific, can be administered intravitreally, and have potential for panretinal effect. Since the approval of the ASO fomivirsen in 1998, there has been great progress in RNA therapy, including siRNA with its high specificity, shRNA, which can be encoded within a transgene and delivered via a viral vector to achieve durability, and ERSGs, which could have broad applicability across mutations. With encouraging early clinical research, RNA therapies may play a key role in the future treatment of IRDs and other retinal disorders. RP
REFERENCES
- Bajan S, Hutvagner G. RNA-based therapeutics: from antisense oligonucleotides to miRNAs. Cells. 2020;9(1):E137.
- Wang S, Koster KM, He Y, Zhou Q. miRNAs as potential therapeutic targets for age-related macular degeneration. Future Med Chem. 2012;4(3):277-287.
- Zhou Q, Gallagher R, Ufret-Vincenty R, Li X, Olson EN, Wang S. Regulation of angiogenesis and choroidal neovascularization by members of microRNA-23~27~24 clusters. Proc Natl Acad Sci U S A. 2011;108(20):8287-8292.
- Shen J, Yang X, Xie B, et al. MicroRNAs regulate ocular neovascularization. Mol Ther. 2008;16(7):1208-1216.
- Sabatel C, Malvaux L, Bovy N, et al. MicroRNA-21 exhibits antiangiogenic function by targeting RhoB expression in endothelial cells. PLoS One. 2011;6(2):e16979.
- Alnylam and the Science of RNAi. Alnylam. 2020. Accessed March 31, 2020. https://www.alnylam.com/our-science/the-science-of-rnai/
- Moore CB, Guthrie EH, Huang MT, Taxman DJ. Short hairpin RNA (shRNA): design, delivery, and assessment of gene knockdown. Methods Mol Biol. 2010;629:141-158.
- Lambeth LS, Smith CA. Short hairpin RNA-mediated gene silencing. Methods Mol Biol. 2013;942:205-232.
- Askou AL, Pournaras JA, Pihlmann M, et al. Reduction of choroidal neovascularization in mice by adeno-associated virus-delivered anti-vascular endothelial growth factor shorthairpin RNA. J Gene Med. 2012;14(11):632-641.
- Cideciyan AV, Sudharsan R, Dufour VL, et al. Mutation-independent rhodopsin gene therapy by knockdown and replacement with a single AAV vector. Proc Natl Acad Sci U S A. 2018;115(36):E8547-E8556.
- Gene Therapy. IVERIC bio. Published 2020. Accessed March 31, 2020. https://ivericbio.com/gene-therapy/#adrp
- Jabs D, Griffiths P. Fomivirsen for the treatment of cytomegalovirus retinitis. Am J Ophthalmol. 2002;133(4):552-556.
- Bennett C, Baker B, Swayze E, Geary R. Pharmacology of antisense drugs. Ann Rev Pharmacol Toxicol. 2017;57:81-205.
- Garanto A, Sangermano R, Albert S, et al. Antisense oligonucleotide-based restoration of ABCA4 splicing defects caused by deep-intronic mutations associated with Stargardt disease. Paper presented at: Association for Research in Vision and Ophthalmology Annual Meeting; April 2018; Honolulu, HI.
- ProQR R&D Day Presentation. January 29, 2019, New York. Data on file with ProQR. Accessed March 31, 2020. https://ir.proqr.com/static-files/a04d2d08-6e89-437a-9057-3d409f6f447e .
- Pipeline - Eloxx Pharmaceuticals. Published 2020. Accessed March 31, 2020. https://www.eloxxpharma.com/pipeline