Retinal surgeons have long utilized the simplest form of nanotechnology without realizing it with intravitreal triamcinolone injections. The microparticulate suspension of triamcinolone is a hydrophobic nanostructure that allows for sustained gradual release of steroids for biological response. Our understanding of nanotechnology has since come a long way.
Nanotechnology is the scientific field on the nanometer scale that leverages chemical and physical properties that are unique to certain nanostructures in application-specific designs. Nanotechnology in medicine is leveraging or enhancing known biological molecular machinery to diagnose and treat diseases. It has gained significant interest because of the ability to engineer unique molecular structures to perform a specific targeted task that otherwise would be very hard to biologically create. Imagine sophisticated building blocks that integrate biology, chemistry, and physics according to your design.
For scale, a cell is typically 10 microns to 50 microns in size and nanoparticles are typically 10 nm to 100 nm in size, approximately a 500-fold magnitude difference. Examples of naturally occurring bionanostructures are antibodies, which have an average radius of 5 nm to 6 nm, and viruses, which range from 10 nm to 200 nm. By appreciating this difference in scale, the power behind nanotechnology is the ability to engineer and design molecular interactions at the protein and cellular machinery level where as current medical treatments are aimed either at a pure molecular drug level interaction or macroscopic tissue-level interaction.
Various known chemical, physical, and biological structures, such as fluorescent proteins, gold or iron oxide nanoparticles, antibodies, and enzymes, can serve as different types of building blocks that can be combined in numerous ways to form novel structures and designed applications. For example, antibodies can be covalently attached to photoabsorptive or radioablative materials, creating a nanoparticle that targets cells for thermal ablative therapy. The same nanoparticle could be then combined with a ferromagnetic material for MRI imaging to create a multifunctional nanoparticle with the ability to visualize targeted cells on MRI imaging while destroying the targeted cells using radiofrequency or photoablative therapies (Figure 1).
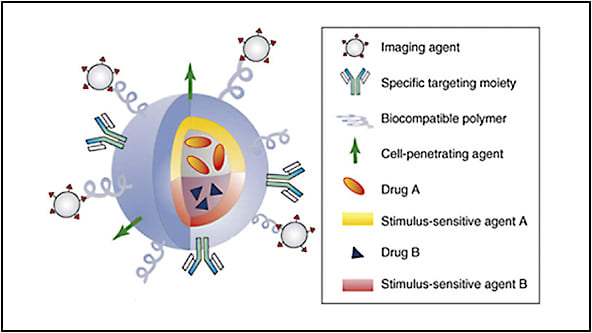
Within the field of retina, nanotechnology has several areas of specific interest that may advance our field in the areas of drug delivery, diagnostic imaging, therapeutic treatments, and nanodevices. This article will briefly summarize those potential applications and developments within our field.
DRUG DELIVERY AND GENE DELIVERY
There is significant interest in designing drug-delivery systems using nanotechnology because of the ability to engineer longer duration drug systems to decrease treatment frequency burden as well as the development of more targeted therapy. By creating targeted drug delivery and gene delivery, unwanted side effects or off-targeting effects can also be mitigated.
Nanoparticles can be designed to encase and protect drugs of interest; they can not only gain access to intracellular environments by endocytosis but also be trafficked to specific areas within the cell through conjugated targeting peptides. A unique property to nanoparticles is in many retinal disease states such as inflammation, angiogenesis, and tumor formation: the leaky vascular state preferentially causes nanoparticles to accumulate due to their inherent size. This then allows for higher drug level states to be achieved when embedded within nanoparticles.1 Furthermore, drugs that are highly water insoluble, such as melphalan, can be embedded in nanostructures that permit higher drug concentrations. This is achieved when the outer structural layer is highly water soluble while the inner nanoparticle structure very hydrophobic to carry the drug. These nanoparticles can improve existing drug stability, durability, and therapeutic effect by increasing drug-carrying capacity.
Another form of delivery system is polymeric nanogels, which use a matrix of hydrophilic polymers to load aqueous soluble drugs and then removal of the solvent to create a compact delivery system where the kinetics of drugs release can be controlled by varying the degree of crosslinking, and hydrophobicity of the polymers. The drug release can be further refined to be temperature or pH controlled. These polymers can be polysaccharide-sugar derivatives such as chitosan or agarose, protein based such as collagen, or amino-acid based such as poly-lactic-glycolic acid which is used in Vicryl sutures (Ethicon, Inc.) and Ozurdex dexamethasone implant (Allergan). By controlling these parameters, a sustained-release system can be created to gradually release drugs of interest.
The primary obstacle is making sure the embedded drug retains its biological activity after synthesis or encapsulation. An example of this potential technology has been shown in a controlled-release bevacizumab PEG-PLGA nanoparticle injected intravitreally in a laser-induced CNV rat model. These nanoparticles allow for prolonged, sustained drug delivery and decrease treatment burden for patients.2
For drugs that may have a very narrow therapeutic window due to cell toxicity but that are highly effective in specific circumstances, drug-embedded nanoparticles can be designed with a release mechanism that responds to light, temperature, ultrasound, pH, and other stimuli while targeting specific types of cells or proteins through conjugated antibodies. These triggers thereby allow a controlled drug delivery only to targeted cells without damage to surrounding tissues. The drug is then released by exposure to the designed trigger after the nanoparticle has bound to specific targets. Similarly, one can imagine how these tools can be used to augment gene therapy by enhancing or improving upon existing viral delivery vectors.
While nanoparticles yield significant promise of new therapies, challenges remain. Nanoparticles can vary greatly in design, charge, biosurface compatibility, and stability when placed in aqueous saline environments. Nanoparticles have the potential to incite inflammation when they aggregate over time depending on surface charges and surface water solubility. In addition, large-scale reproducible manufacturing has proven challenging for nanoparticles because of the sensitivity to environmental parameters during synthesis.
DIAGNOSTIC IMAGING
Fluorescein and indocyanine-green angiography and OCT, although extensively used in retina to diagnose diseases, are monomodal and dye-limited. The physics behind certain nanostructures and nanoparticles are very interesting because optical response can be tuned based on their size. For example, nanoshells and nanorods can absorb and scatter light and can be used as a phototherapeutic tool in destroying targeted cells when designed to absorb specific wavelengths of light or to improve diagnostic imaging as a contrast agent when designed to scatter light. The wavelengths in which the nanoparticle interacts with light are directly proportional to the nanoparticle’s size and shape.
Similarly, quantum dots are nanoparticles that fluoresce with a very high quantum yield (the conversion efficiency of excited light in generating a fluorescent signal, many fold greater than any fluorescent dye) and are tunable to any desired wavelength of fluorescence based on their size. However, some of these fluorescent nanostructures, such as quantum dots, may contain toxic elements such as cadmium, making it unlikely to ever be used in humans. Zinc-based quantum dots are a potentially viable alternative; however, their blue-wavelength fluorescence limits in vivo applications due to increased scattering that causes signal attenuation.
An alternative strategy that researchers are investigating is embedding biologically safe fluorescent dye molecules within biocompatible nanoparticles of specific sizes to enhance brightness and stability. Not only will this facilitate stronger fluorescent signals but also it will allow researchers to evaluate the permeability of different-sized nanoparticles by altering the nanoparticle size. Different nanoparticle sizes would fluoresce at differing wavelengths. Angiography would then become a multispectral imaging tool, offering the ability to discern different vascular permeability states based on fluorescent color patterns since each permeability state would effectively show a differing unique fluorescent wavelength. We would then be able to evaluate in vivo how permeability changes in disease progression, such as in DME, in addition to how it is modulated with anti-VEGF and steroid treatment.
One could extend the concept of conjugating fluorescent nanoparticles to different antibodies or peptides that would target specific protein expression. Each targeted biomarker (protein) would have its own unique fluorescent wavelength, permitting an entirely new way to evaluate in vivo biological protein expression of multiple proteins of interest simultaneously and how they may interact. We would be able to optically barcode the protein expressions on the retinal surface with multispectral fluorescent analysis.
This would be an entirely novel way of functionally assessing protein expression within the retina and truly evaluating biomarkers instead of clinical surrogate biomarkers of OCT/FA response to specific treatment paradigms. Physicians could evaluate protein expression profiles within the retina of a patient and design a tailored therapy based on that protein expression.
These concepts can also be applied to OCT as many OCT-based nanoparticle contrast agents have also been identified such as gold-based nanoshells, nanorods, and nanocages.3 These gold nanoparticles exhibit unique scattering coefficients that can be tuned to OCT wavelengths and conjugated to targeting antibodies. These contrast agents can be used to enhance weak OCT signals to improve visualization of the outer choroid or be adapted through targeting moieties to provide information beyond structural findings. Several of these gold nanoparticles have been studied and found to be biocompatible and safe and may one day be potentially used to allow the use of OCT to evaluate protein expression in disease states beyond simply structural information.
Other imaging modalities have also been explored, such as MRI-based contrast agents. Engineered magnetic hybrid nanoparticles provide 4x improved signal strength compared to standard Gd-based contrast agents and can be coloaded with hydrophobic therapeutic compounds inside the nanoparticle core for a theranostic system.4
THERAPEUTIC MODALITIES AND TREATMENTS
Targeted photothermal applications have also been developed with gold nanoparticles designed to be predominantly absorptive in light energy instead of scattering (which would benefit OCT diagnostic technologies). These nanoparticles would absorb light energy at specific wavelengths, generate heat, and thermally damage targeted cells, such as an intraocular melanoma or retinoblastoma or a CNV lesion, as long as a unique biomarker can be identified.
The tremendous advantage with targeted nanotherapy would be the ability to destroy cells of interest with minimal surrounding tissue damage. Similarly, nanostructures have been developed that can respond to radiofrequencies or magnetic resonance to generate heat and thermally ablate targeted proteins/cells of interest. The ability to easily modify the nanoparticles or conjugate nanostructures with a treatment moiety has created a rapidly growing field of theranostic applications, or modalities that can simultaneously diagnose and treat diseases.
NANODEVICES AND ARTIFICIAL PHOTORECEPTOR TECHNOLOGIES
Artificial retinal technologies have made incredible strides with microelectronic devices such as the Argus II retinal implant (Second Sight) and a photovoltaic subretinal implant developed at Stanford University.5 Work is currently ongoing in developing photoreceptor implants on a nano scale by leveraging the photoresponsive behavior of silicon materials in generating current.6 Alternative designs have also looked at photoconducting polymers coupled to nanowires such as carbon nanotubes that are neuroadhesive and have demonstrated the ability to stimulate light-insensitive chick retinas.7
The advantage in these carbon nanotube-based photoresponsive structures is the potential for cellular spatial image resolution, the ability to be powered by inherent nanodevice electrons and incoming photons eliminating the need for an external power source, and improved biocompatibility without concerns of metallic electrode oxidation or thermal tissue damage which are parameters that need to be considered in the design of microelectronic devices because of the macro scale of the technology.
FUTURE APPLICATIONS
The future of nanotechnology in retina has many promising avenues of exploration and application. As our understanding of various nanomaterials improves and their biocompatibility and targeting is refined, we will have new tools and therapies not only to diagnose diseases at earlier states but also to restore sight and treat diseases that currently have no means of visual restoration. RP
REFERENCES
- Singh SR, Grossniklaus, HE, Kang, SJ, Edelhauser, HF, Ambati, BK, Kompella UB. Intravenous trasnferrin, RGD peptide and dual-targeted nanoparticles enhance anti-VEGF intraceptor gene delivery to laser-induecd CNV. Gene Ther. 2009;16(5):645-659.
- Pan CK, Durairaj C, Kompella UB, et al. Comparison of long-acting bevacizumab formulations in the treatment of choroidal neovascularization in a rat model. J Ocul Pharmacol Ther. 2011;27(3):219-224.
- Pierce, MC, Javier, DJ, Richards-Kortum, R. Optical contrast agents and imaging systems for detection and diagnosis of cancer. Int J Cancer. 2008;123(9):1979-1990.
- Aryal S, Key J, Stigliano C, Ananta JS, Zhong M, Decuzzi P. Engineered magnetic hybrid nanoparticles with enhanced relaxivity for tumor imaging. Biomaterials. 2013;34(31):7725-7732.
- Mathieson K, Loudin J, Goetz G, et al. Photovoltaic retinal prosthesis with high pixel density. Nat Photonics. 2012;6(6):391-397.
- Khraiche ML, Lo Y, Wang D, Cauwenberghs G, Freeman W, Silva GA. Ultra-high photosensitivity silicon nanophotonics for retinal prosthesis: electrical characteristics. Conf Proc IEEE Eng Med Biol Soc. 2011;2933-2936.
- Bareket L, Waiskopf N, Rand D, et al. Semiconductor nanorod-carbon nanotube biomimetic films for wire-free photostimulation of blind retinas. Nano Lett. 2014;14(11):6685-6692.