The Uses of Retinal Imaging In the Management Of Neurological Disorders
In addition to patients with diabetic retinopathy, those with Alzheimer’s and other diseases can benefit.
MARIANNE PHILLIPS, IBSC • TIMOTHY WONG, IBSC • SHEREEN NIZARI, BSC, • M. FRANCESCA CORDEIRO, MD
Increasing evidence has emerged that the health of retinal neurones reflects that of neurones in the brain (Figure 1).1 Being able to accurately image the retina may be of interest in potentially diagnosing and following up many neurodegenerative diseases.
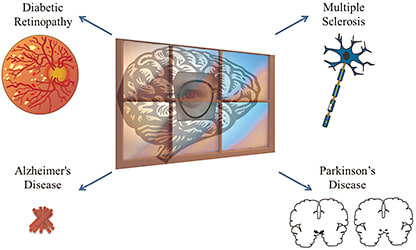
Figure 1. A drawing depicting how ocular changes in diabetic retinopathy, multiple sclerosis, Alzheimer’s disease, and Parkinson’s disease can all be used as a “window to the brain.”
Key diseases considered in this review include multiple sclerosis, Parkinson’s disease (PD), Alzheimer’s disease (AD), and diabetic retinopathy, alongside an outline and evaluation of the various existing imaging techniques.
CURRENT RETINAL IMAGING METHODS
Fundus Autofluorescence
Fundus autofluorescence is a noninvasive imaging method that uses the properties of retinal inherent autofluorescence to identify pathological changes and disease states of the retina.
The scanning laser ophthalmoscope has become increasingly popular for fundus AF imaging and has been advocated for diseases such as geographic atrophy secondary to AMD.
Fluorescein Angiography and Indocyanine Green Angiography
Both FA and ICGA are administered intravenously to diagnose abnormal retinal disease in patients. Images are acquired at regular intervals after injection, using the fluorescent settings on either the fundus camera or the SLO.
Marianne Phillips, IBSC, and Timothy Song, IBSc, are medical students at University College London (UCL). Shereen Nizari, BSc, is a research assistant/technician at UCL. M. Francesca Cordeiro, MD, is professor of retinal neurodegeneration and glaucoma studies at UCL. None of the authors reports any financial interests in products mentioned in this article. Prof. Cordeiro can be reached at m.cordeiro@ucl.ac.uk.
An important difference between the dyes is their binding to proteins: more than 98% of ICG is protein-bound, compared to FA, which only binds to approximately 60% to 80%, making the latter leak rapidly. FA provides detailed images of retinal capillaries, whereas ICG highlights choroidal vasculature.
Fluorescein angiography hyperfluorescence indicates leaks or excessive vessels (proliferative diabetic retinopathy, “wet” AMD), and it is particularly useful for diagnosing macular edema). ICG is useful for CNV. The side effects of FA include dizziness and nausea, with a risk of anaphylaxis.
Optical Coherence Tomography
An imaging system based on low-coherence interferometry, in which changes in reflectivity occur at very high axial resolution, OCT emits light to a reference (moving at a set distance and frequency) and sample (the retina) and then back toward a detector. Demodulation and interpretation are then applied to create an image of the sample structure, compared to the reference.2
For example, a high degree of sample cell/retinal thickness means a greater distance for light to pass through than normal tissue, and more time needed for its return (distance = speed x time). This method produces high-resolution 3D images (via spectral-domain OCT) of a patient’s retinal morphology and density.
Scanning Laser Ophthalmoscopy
Scanning laser ophthalmoscopy uses an emitted beam redirected by oscillating mirrors to obtain two-dimensional images reflected from the retina and optic disc. A luminance detector measures the amount of light reflected from each image after passing through the confocal aperture. Shifting the confocal aperture allows for the acquisition of multiple sections through the tissue.
Adaptive Optics, Scanning Laser Polarimetry, and High-resolution Reflectance Imaging
Based on astronomy telescopes, adaptive optics corrects for different aberrations using a patterned guide laser to sense errors in the optics of the eye and a deformable mirror to correct for them in real time.
Scanning laser polarimetry is a confocal imaging system with a polarimeter to measure the birefringence the retinal nerve fiber layer (RNFL) causes. High-resolution reflectance imaging, based around a fundus camera with a high-quality charge-coupled device camera, can take a rapid sequence of images that can measure wavelength-dependent reflectance changes with very high temporal resolution.
Detection of Apoptosing Retinal Cells
This method can quantify the number of apoptosing retinal cells (particularly retinal ganglion cells — RGCs) at a specific moment in time in vivo (Figure 2, page 50). With patient follow-up, it could potentially help to identify abnormal activity, monitor disease progression, and assess therapy.
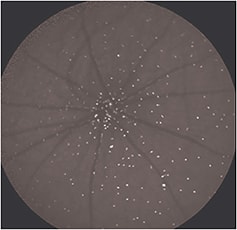
Figure 2. An example of an image acquired using the DARC technique in a rat model, in which fluorescently labeled Annexin marks retinal ganglion cells undergoing apoptosis in vivo, each cell identified by a white spot.
The technique uses fluorescently tagged Annexin-V, injected intravenously, to identify cells with externalized phosphatidylserine.3 We can then count these cells to assess the current level of apoptosis. Phase 1 clinical trials are due to begin shortly to establish safety and proof of concept in patients.3-5
MULTIPLE SCLEROSIS
Multiple sclerosis is a neurodegenerative disease affecting more than 1.3 million people worldwide. It occurs due to an aberrant immune process, causing antibodies and inflammatory factors to form against myelin-related proteins, resulting in the demyelination of CNS neurones.
Magnetic resonance imaging scans show diagnostic lesions within the brain, and corresponding muscle groups may show weakness in acute phases or progressively, depending on the subtype of MS.
Loss of sensation and autonomic control and Charcot’s triad of cerebellar symptoms — nystagmus, dysarthria, and intention tremor — may also occur. Additionally, 80% of MS patients experience visual impairment, with 25% to 50% of first-reported MS episodes being optic neuritis (ON).6-8 Reports have detailed strong correlations among visual loss, RNFL thinning (measured by OCT), and disability in MS patients.9
Study Data From Patients With MS
Studies on cohorts of MS patients (with and without ON) have measured RNFL thickness with OCT. Findings have indicated thinner RNFL thickness in MS patients compared to controls, with the greatest thinning in those with ON.9-12 These studies, alongside another study directly testing OCT reproducibility,8 indicate that OCT is reliable for assessing retinal changes in MS.
Additionally, OCT has helped to elucidate some of the mechanisms of this disease, showing that ON alone is not evidence of RNFL thinning or visual disturbance.11 Components of MS now include possible retrograde degeneration or antibodies against antigens other than myelin-related proteins, because the RNFL has unmyelinated axons that the disease also affects. Such results indicate how the eye can be a window to understanding more about pathology in the brain.
Overall, OCT provides high-resolution images of the RNFL, resembling histological images previously obtained from MS patients post mortem.7,13 Results have also reflected MRI and visual reports for MS patients.14 Future follow-up studies, with larger cohorts, will be necessary to understand whether OCT is useful in monitoring MS progression and therapy efficacy.7
Some trials are already under way, such as NCT01371071 and NCT01705236 (from ClinicalTrials.gov). Issues to explore include whether the RNFL continues to thin with time (in MS) and if it still correlates with the degree of disability, as well as whether therapies can slow or prevent this loss.
PARKINSON’S DISEASE
Parkinson’s disease is the second most common neurodegenerative disorder after Alzheimer’s disease.15 Parkinson’s is primarily a progressive motor disorder associated with degeneration of dopaminergic neurons in the substantia nigra.
Research has shown Parkinson’s to be a multisystem disorder with additional nonmotor impairments and pathology occurring outside the basal ganglia. Dopamine plays a major role in motor function, but it is also an important neurotransmitter/neuromodulator in the retina.
Overt Parkinson’s disease does not present until roughly 80% of dopaminergic neuronal loss has occurred in the striatum. This neuronal loss has also been found outside the substantia nigra, including in the RGCs.
Visual Areas Affected by Parkinson’s
Higher visual areas also contain dopaminergic neurons that Parkinson’s may affect. As such, visual symptoms are of particular interest. Visual functions, such as absolute and temporal sensitivity, color vision, and spatial contrast sensitivity, are impaired in Parkinson’s.16-18
Such changes may be due to structural alterations in the retina, which retinal imaging detects. Studies using OCT have found that greater RNFL thinning occurs in Parkinson’s disease patients at specific sites, compared to controls.19,20
Even more recently, researchers have reported changes in OCT parameters in a Parkinson’s disease model.21,22 Dopamine establishes the gain of RGCs and modulates the receptive fields of RGCs through D1 and D2 receptors.
Recent Research
Inzelberg et al19 showed that the reduction in sensitivity in these visual fields and the RNFL thinning sites matched topographically, suggesting localized nerve fiber defects.
In addition, Atlintas et al20 suggested an inverse correlation existed between the severity of Parkinson’s and inner foveal thickness.
Increasing evidence has suggested a correlation between visual problems and progressive motor disability in Parkinson’s. However, large-scale studies are under way to further elucidate the link between the structural and clinical findings at different stages of PD (NCT01928212). Nevertheless, confounding treatment effects pose a problem in monitoring such retinal changes.
ALZHEIMER’S DISEASE
Alzheimer’s disease is the most common progressive neurodegenerative disease and cause of dementia in the elderly.23 It has a global prevalence of 36 million, and researchers estimated that it will double every 20 years to reach 115 million in 2050.24
The pathological hallmarks of Alzheimer’s include the deposition of extracellular plaques of ß-amyloid (Aß) fragments, as well as hyperphosphorylated tau protein (p-tau), which can form intracellular neurofibrillary tangles (NFTs).25 This process leads to extensive neuronal cell damage and death, resulting in cognitive decline. Alzheimer’s patients tend to have visual problems that affect visual acuity, color vision, depth perception, and spatial contrast sensitivity.
Despite earlier studies indicating that these problems were due to nerve degeneration in the visual cortex, some researchers have proposed that pathology in the retina and optic nerve also plays an important role. For example, an autopsy study of Alzheimer’s patients revealed decreased numbers of RGCs and axons.26
Findings From Animal Models
Increasing evidence has shown that the retina can reliably reflect pathology in the brains of patients with Alzheimer’s. APPswe and Presenilin (PS) 1ΔE9 transgenic mice carrying the genes that cause early-onset familial Alzheimer’s disease in humans developed human Aß deposits in their retinas at advanced stages of the disease.27,28
In fact, Koronyo-Hamaoui et al (2011) provided evidence that Aß plaques form in the retina before they manifest in the brain. Garcia-Allonza et al29 used curcumin, a natural fluorochrome, to bind and label Aß plaques in live AD mice, allowing for in vivo imaging of Aß plaques in the retinas. The average plaque size measured in vivo was comparable to that measured by immunostaining ex vivo.
The use of OCT in 22 patients with Alzheimer’s revealed RNFL thinning at the superior and inferior quadrants, compared to 22 controls.30 Furthermore, DARC imaging was able to detect RGC death in the retinas of live Alzheimer’s mouse models. This noninvasive technique also allows for the direct tracking and imaging of the progression of amyloid pathology.31
The diagnosis of Alzheimer’s is a complicated and difficult process, one that could potentially improve with the identification of Alzheimer’s pathology in the retina, even before clinical symptoms manifest.
DIABETIC RETINOPATHY
The increasing prevalence of diabetes and its associated eye diseases, including DR, means it is the leading cause of blindness in patients younger than age 65 years.32,33.
Diabetic retinopathy presents with vascular and neuronal alterations in the retina, as a result of chronically high glucose concentrations that form advanced glycosylation end products. These concentrations can damage the basement membranes and can produce DME or proliferative DR, in which new abnormal vessels form and can bleed easily.34
Early on, DR is asymptomatic, but finding it at this stage is vitally important because suitable interventions (diet changes and treating hypertension) can prevent vision loss and resulting disability (and related health-care expenses).35,36
Early Diagnosis of DR and Imaging Issues
We can achieve such early diagnosis with direct ophthalmoscopy — examining the vasculature especially — although mydriatic fundus photography has greater sensitivity.37,38 Additionally, for DME, OCT has greater sensitivity than stereoscopic fundus photography.39-41
We must still be cautious in interpreting OCT of RNFL thickness, because researchers have found variability between machine subtypes.42,43 In contrast, OCT measurements from the same machine have proved reproducible and repeatable.44,45
Interestingly, evidence has suggested that retinal neurones degenerate early on in DR, and such aberrant changes may even begin before microcirculatory changes.46,47
Standard fundus photography and ophthalmoscopy are inadequate for detecting such subtle neuronal changes, so OCT may be of interest as an adjunct to obtain objective, quantifiable retinal thickness measurements.
We can then monitor these measurements over time and, importantly, determine whether proposed anti-VEGF treatments are effective in reducing not only vascular proliferation and leakage but also retinal neuronal loss.36
CONCLUSION
The eye may act as a window to the brain, with retinal pathology identified early on in multiple neurodegenerative disorders. The retinal imaging techniques discussed in this review provide noninvasive methods for detecting and monitoring such pathologies, with high resolution and sensitivity — notably with OCT.
Larger progressive studies are necessary, however, to determine the reliability of these tools in diagnosing and monitoring neurodegenerative diseases. RP
REFERENCES
1. Frohman EM, Fujimoto JG, Frohman TC, Calabresi PA, Cutter G, Balcer LJ. Optical coherence tomography: a window into the mechanisms of multiple sclerosis. Nat Clin Pract Neurol. 2008;4:664-675.
2. Schuman JS, Hee MR, Arya AV, et al. Optical coherence tomography: a new tool for glaucoma diagnosis. Curr Opin Ophthalmol. 1995;6:89-95.
3. Cordeiro MF, Migdal C, Bloom P, Fitzke FW, Moss SE. Imaging apoptosis in the eye. Eye (Lond). 2011;25:545-553.
4. Normando EM, Turner LA, Cordeiro MF. The potential of annexin-labelling for the diagnosis and follow-up of glaucoma. Cell Tissue Res. 2013;353:279-285.
5. Garcia-Valenzuela E, Shareef S, Walsh J, Sharma SC. Programmed cell death of retinal ganglion cells during experimental glaucoma. Exp Eye Res. 1995;61:33-44.
6. Warner J, Lessell S. Neuro-ophthalmology of multiple sclerosis. Clin Neurosci. 1994;2:180-188.
7. Frohman E, Costello F, Zivadinov R, et al. Optical coherence tomography in multiple sclerosis. Lancet Neurol. 2006;5:853-863.
8. Cettomai D, Pulicken M, Gordon-Lipkin E, et al. Reproducibility of optical coherence tomography in multiple sclerosis. Arch Neurol. 2008;65:1218-1222.
9. Walter SD, Ishikawa H, Galetta KM, et al. Ganglion cell loss in relation to visual disability in multiple sclerosis. Ophthalmology. 2012;119:1250-1257.
10. Trip SA, Schlottmann PG, Jones SJ, et al. Retinal nerve fiber layer axonal loss and visual dysfunction in optic neuritis. Ann Neurol. 2005;58:383-391.
11. Fisher JB, Jacobs DA, Markowitz CE, et al. Relation of visual function to retinal nerve fiber layer thickness in multiple sclerosis. Ophthalmology. 2006;113:324-332.
12. Pulicken M, Gordon-Lipkin E, Balcer LJ, Frohman E, Cutter G, Calabresi PA. Optical coherence tomography and disease subtype in multiple sclerosis. Neurology. 2007;69:2085-2092.
13. Xue K, Hildebrand GD. Retinal imaging: what the neurologist needs to know. Pract Neurol. 2013;13:236-244.
14. Grazioli E, Zivadinov R, Weinstock-Guttman B, et al. Retinal nerve fiber layer thickness is associated with brain MRI outcomes in multiple sclerosis. J Neurol Sci. 2008;268:12-7.
15. de Lau LM, Breteler MM. Epidemiology of Parkinson’s disease. Lancet Neurol. 2006;5:525-535.
16. Djamgoz MB, Hankins MW, Hirano J, Archer SN. Neurobiology of retinal dopamine in relation to degenerative states of the tissue. Vis Res. 1997;37:3509-3529.
17. Bodis-Wollner I. Visual deficits related to dopamine deficiency in experimental animals and Parkinson’s disease patients. Trends Neurosci. 1990;13:296-302.
18. Jackson GR, Owsley C. Visual dysfunction, neurodegenerative diseases, and aging. Neurol Clin. 2003;21:709-728.
19. Inzelberg R, Ramirez JA, Nisipeanu P, Ophir A. Retinal nerve fiber layer thinning in Parkinson disease. Vis Res. 2004;44:2793-2797.
20. Altintas O, Iseri P, Ozkan B, Caglar Y. Correlation between retinal morphological and functional findings and clinical severity in Parkinson’s disease. Doc Ophthalmol. 2008;116:137-146.
21. Kirbas S, Turkyilmaz K, Tufekci A, Durmus M. Retinal nerve fiber layer thickness in Parkinson disease. J Neuroophthalmol. 2013;33:62-65.
22. Satue M, Garcia-Martin E, Fuertes I, et al. Use of Fourier-domain OCT to detect retinal nerve fiber layer degeneration in Parkinson’s disease patients. Eye (Lond). 2013;27:507-514.
23. Goedert M, Spillantini MG. A century of Alzheimer’s disease. Science. 2006;314:777-781.
24. Wortmann M. Dementia: a global health priority - highlights from an ADI and World Health Organization report. Alzheimers Res Ther. 2012;4:40.
25. Hardy J. A hundred years of Alzheimer’s disease research. Neuron. 2006;52:3-13.
26. Hinton DR, Sadun AA, Blanks JC, Miller CA. Optic-nerve degeneration in Alzheimer’s disease. N Engl J Med. 1986;315:485-487.
27. Ning A, Cui J, To E, Ashe KH, Matsubara J. Amyloid-beta deposits lead to retinal degeneration in a mouse model of Alzheimer disease. Invest Ophthalmol Vis Sci. 2008;49:5136-5143.
28. Perez SE, Lumayag S, Kovacs B, Mufson EJ, Xu S. Beta-amyloid deposition and functional impairment in the retina of the APPswe/PS1DeltaE9 transgenic mouse model of Alzheimer’s disease. Invest Ophthalmol Vis Sci. 2009;50:793-800.
29. Garcia-Alloza M, Borrelli LA, Rozkalne A, Hyman BT, Bacskai BJ. Curcumin labels amyloid pathology in vivo, disrupts existing plaques, and partially restores distorted neurites in an Alzheimer mouse model. J Neurochem. 2007;102:1095-1104.
30. Lu Y, Li Z, Zhang X, et al. Retinal nerve fiber layer structure abnormalities in early Alzheimer’s disease: evidence in optical coherence tomography. Neurosci Lett. 2010 Aug 9;480:69-72.
31. Cordeiro MF, Guo L, Coxon KM, et al. Imaging multiple phases of neurodegeneration: a novel approach to assessing cell death in vivo. Cell Death Dis. 2010;1:e3.
32. Mackenzie S, Schmermer C, Charnley A, et al. SDOCT imaging to identify macular pathology in patients diagnosed with diabetic maculopathy by a digital photographic retinal screening programme. PLoS One. 2011;6:e14811.
33. Simo R, Hernandez C. Neurodegeneration is an early event in diabetic retinopathy: therapeutic implications. Br J Ophthalmol. 2012;96:1285-1290.
34. Fowler MJ. Microvascular and macrovascular complications of diabetes. Clin Diabet. 2008 April 1, 2008;26:77-82.
35. Arun CS, Al-Bermani A, Stannard K, Taylor R. Long-term impact of retinal screening on significant diabetes-related visual impairment in the working age population. Diabet Med. 2009;26:489-492.
36. Antonetti DA, Klein R, Gardner TW. Diabetic retinopathy. N Engl J Med. 2012;366:1227-1239.
37. Harding SP, Broadbent DM, Neoh C, White MC, Vora J. Sensitivity and specificity of photography and direct ophthalmoscopy in screening for sight threatening eye disease: the Liverpool Diabetic Eye Study. BMJ. 1995;311:1131-1135.
38. Hutchinson A, McIntosh A, Peters J, et al. Effectiveness of screening and monitoring tests for diabetic retinopathy--a systematic review. Diabet Med. 2000;17:495-506.
39. Moreira RO, Trujillo FR, Meirelles RM, Ellinger VC, Zagury L. Use of optical coherence tomography (OCT) and indirect ophthalmoscopy in the diagnosis of macular edema in diabetic patients. Int Ophthalmol. 2001;24:331-336.
40. Virgili G, Menchini F, Dimastrogiovanni AF, et al. Optical coherence tomography versus stereoscopic fundus photography or biomicroscopy for diagnosing diabetic macular edema: a systematic review. Invest Ophthalmol Vis Sci. 2007;48:4963-4973.
41. Mackenzie S, Schmermer C, Charnley A, et al. SDOCT imaging to identify macular pathology in patients diagnosed with diabetic maculopathy by a digital photographic retinal screening programme. PLoS One. 2011;6:e14811.
42. Giani A, Cigada M, Choudhry N, et al. Reproducibility of retinal thickness measurements on normal and pathologic eyes by different optical coherence tomography instruments. Am J Ophthalmol. 2010;150:815-824.
43. Bentaleb-Machkour Z, Jouffroy E, Rabilloud M, Grange JD, Kodjikian L. Comparison of central macular thickness measured by three OCT models and study of interoperator variability. ScientificWorldJournal. 2012;2012:842795.
44. Forooghian F, Cukras C, Meyerle CB, Chew EY, Wong WT. Evaluation of time domain and spectral domain optical coherence tomography in the measurement of diabetic macular edema. Invest Ophthalmol Vis Sci. 2008;49:4290-4296.
45. Fiore T, Androudi S, Iaccheri B, et al. Repeatability and reproducibility of retinal thickness measurements in diabetic patients with spectral domain optical coherence tomography. Curr Eye Res. 2013;38:674-679.
46. Villarroel M, Ciudin A, Hernandez C, Simo R. Neurodegeneration: An early event of diabetic retinopathy. World J Diabetes. 2010;1:57-64.
47. Stem MS, Gardner TW. Neurodegeneration in the pathogenesis of diabetic retinopathy: molecular mechanisms and therapeutic implications. Curr Med Chem. 2013;20:3241-3250.