Imaging the Evolution of Dry AMD
Advancements in imaging have resulted in greater ability to diagnose and perhaps treat dry AMD.
MARCO A. ZARBIN, MD, PhD • PHILIP J. ROSENFELD, MD, PhD
By improving our ability to image the different pathophysiological features of AMD, we will improve our ability to monitor disease progression clinically and to develop novel clinical trial endpoints for monitoring treatment outcomes.1
As our AMD imaging strategies improve, and we begin to target treatment at earlier stages of the disease, we should be able to design better clinical trial endpoints to test efficacy at these earlier stages of AMD.
By targeting earlier stages, we will preserve far more vision than current interventions, which target the later stages of disease, such as geographic atrophy and choroidal neovascularization.
In this article, we review the use of modern imaging technology to visualize the early stages of AMD and to track AMD progression to GA, a topic we have considered in detail elsewhere.2
GEOGRAPHIC ATROPHY
Historically, we have monitored the size and extent of drusen, as well as the presence of pigmentary abnormalities and evidence of GA, using fundus reflectance imaging and autofluorescence imaging. These imaging strategies have been the traditional benchmark by which AMD severity has been graded.3-7
Geographic atrophy, which is the result of retinal pigment epithelium atrophy and atrophy of the underlying choriocapillaris and overlying photoreceptors, has been the typical feature of advanced dry AMD that we have monitored in dry AMD clinical trials.
Marco A. Zarbin, MD, PhD, FACS, is professor and chair of the Institute of Ophthalmology and Visual Science, Rutgers New Jersey Medical School. Philip J. Rosenfeld, MD, PhD, is professor of ophthalmology at the Bascom Palmer Eye Institute at the University of Miami Miller School of Medicine in Florida. Dr. Zarbin reports no financial interests in any products mentioned in this article. Dr. Rosenfeld reports financial interest in Acucela. This research was supported in part by the New Jersey Lions Eye Research Foundation. Dr. Zarbin’s e-mail is zarbin@rutgers.edu.
The effects of treatment on the enlargement of GA have been followed as GA spreads around and toward the foveal center. However, newer imaging technologies are more likely to improve our ability to identify and measure changes at even earlier stages of AMD and to provide improved automated strategies for following disease progression, compared with these conventional methods.8-10
Disease Pathogenesis and Imaging
Early stages of AMD pathogenesis have been associated with several processes, such as oxidative damage, RPE lipofuscin accumulation, and biochemical and structural changes in Bruch’s membrane.11
Eventually, these relatively early and clinically occult changes produce the early clinical findings associated with dry AMD, which include drusen, areas of RPE hyperplasia, and areas of RPE depigmentation.
Spectral-domain OCT systems and swept-source (SS)-OCT, with or without confocal scanning laser ophthalmoscopy, have allowed us to generate simultaneous recordings of cross-sectional OCT images with en face images.
These images are generated using SD-OCT/SS-OCT alone or integrated with short-wavelength fundus autofluorescence (FAF) (blue incident light), confocal fluorescein angiography (FA), indocyanine green angiography, near infrared autofluorescence (NIA), adaptive optics scanning laser ophthalmoscopy (AOSLO), and near infrared reflectance (NIR) imaging.
The different degrees to which light of different wavelengths penetrates tissue accounts for the ability of short-wavelength (blue), intermediate-wavelength (green), long-wavelength (red), and even longer-wavelength light (SD-OCT and SS-OCT) to image different structures in the ocular fundus (Figure 1, page 32).
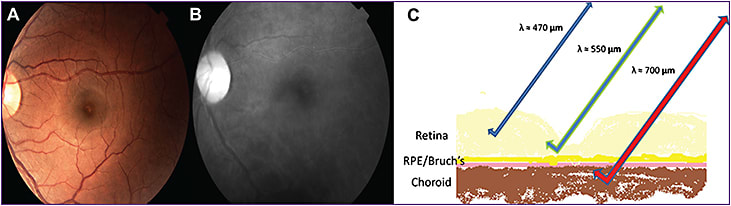
Figure 1. Color fundus photograph (A) of a left eye. Near infrared image (B) with diminished epiretinal details and enhanced subretinal features. Retinal cross-section (C) depicting the capacity of each wavelength to penetrate the retinal layers.
CREDIT: ELSEVIER
The alignment of the cross-sectional OCT images with en face images using high-resolution OCT (5-µm axial resolution) enables us to establish near histological correlation of clinical findings with various features of human pathology.
A Number of Imaging Technologies
Several technologies have enabled us to image some of the pathological changes in patients with dry AMD. Mitochondrial flavoprotein autofluorescence, for example, enables the imaging of mitochondrial oxidative damage.
Spectral-domain OCT and SS-OCT enable the visualization of some of the changes in Bruch’s membrane. Drusen can be imaged with OCT, blue FAF, NIA, FA, and AOSLO. SD-OCT, SS-OCT, and adaptive optics SLO can image photoreceptors, as well as the RPE. GA can be visualized with a variety of modalities, including color fundus photography, OCT, FAF, NIA, FA, and NIR.
Structure-function correlation studies can be undertaken by combining these technologies with microperimetry. We will consider each of these modalities in greater detail in the second half of this review.
IMAGING OXIDATIVE DAMAGE
The mitochondria are the major source of reactive oxygen species in most cells. In the human RPE, for example, phagocytosis of outer segments produces reactive oxygen species and mitochondrial DNA damage.12
Mitochondria are at high risk for oxidative damage.13 Mitochondrial DNA may be among the most important targets of oxidative damage in mitochondria.14 AMD is associated with decreased mitochondrial number and area and with loss of cristae and matrix density,15 increased RPE mitochondrial DNA damage, and decreased repair, as well as decreased mitochondrial respiration.13
Furthermore, some mitochondrial DNA haplogroups are protective against AMD (eg, haplogroup H), whereas others increase one’s risk (eg, haplogroup J).16 One of the mutations strongly associated with increased risk of AMD may be a protein that localizes to the outer mitochondrial membrane.17 RPE mitochondrial damage is associated with reduced metabolic activity (eg, reduced outer segment phagocytosis efficiency)18 and/or apoptosis.
Cellular-level Imaging
Within the mitochondria, flavin adenine dinucleotide (FAD) is bound to succinate dehydrogenase, the second protein complex in the oxidative phosphorylation chain. In its fully oxidized form, FAD accepts two electrons and protons to become FADH2.
As oxidative phosphorylation proceeds in the tricarboxylic acid cycle, FADH2 is oxidized to FAD and generates 1.5 equivalents of adenosine triphosphate (ATP).19 Flavin adenine dinucleotide is significantly more fluorescent than its reduced form, FADH2.20
The physician can follow the changes in the redox state of neural tissue by monitoring flavoprotein fluorescence.21 Cells with stressed mitochondria (eg, preapoptotic cells) exhibit impaired electron transport and increased amounts of FAD, which absorbs blue light and emits green autofluorescence.
Some Research Results
Reinert et al21 reported that flavoprotein autofluorescence signals provide a powerful tool to monitor neuronal activity in vivo and its relationship with mitochondrial metabolism.
Elner et al22 measured retinal flavoprotein autofluorescence in patients with AMD using a fundus camera modified with 467-nm excitation and 535-nm emission filters and a back-illuminated, electron-multiplying, charge-coupled device camera interfaced with a computer equipped with customized image capture software. They imaged a 3° field.
Retinal flavoprotein autofluorescence was increased significantly in AMD patients, as well as in cultured human RPE exposed to oxidative stress (Figure 2).
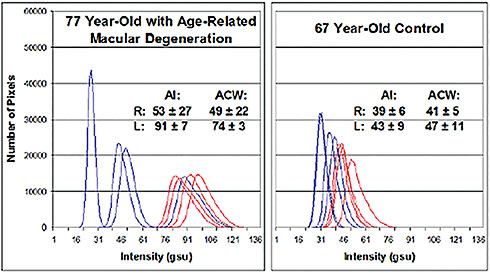
Figure 2. Retinal flavoprotein autofluorescence pixel intensity histograms from a 77-year-old patient with AMD (left) and from a 67-year-old control patient without visible clinical findings of AMD (right). The AMD patient had a history of resolved exudative AMD with BCVA of 20/50 OD and dry AMD with GA and BCVA of 20/400 OS. The figure presents four histograms of the right (blue) and left (red) eye from each patient. Right shift of histograms denotes increased retinal flavoprotein autofluorescence intensity. Broader curve width denotes greater cellular variability in flavoprotein autofluorescence intensity within the imaged 3° field. (AI, average pixel intensity; ACW, average curve width; gsu, grayscale units.)
CREDIT: AMERICAN OPHTHALMOLOGICAL SOCIETY
IMAGING LIPOFUSCIN
With aging, lipofuscin accumulates in RPE cells.23 Outer segment tips are phagocytosed by RPE apical villous processes daily. Phagosomes fuse with lysosomes to form secondary lysosomes in the RPE cytoplasm.
Incomplete digestion of the material contained in phagolysosomes causes formation of lipofuscin granules that may or may not have a melanin core. When illuminated with relatively short-wavelength light, RPE lipofuscin fluoresces.
Researchers have examined the origin of lipofuscin fluorescence intensively. In the outer segment discs, ethanolamine (derived from phosphatidyl ethanolamine in the disc membrane) combines with two retinaldehyde molecules (derived from dissociation of opsin and trans-retinal) to form N-retinyledene-N-retinylethanolamine (A2E). A2E, a bis-pyridinium, is one of the major fluorophores in lipofuscin that causes reactive oxygen species production.24 Excessive RPE lipofuscin accumulation may play an important role in the pathology of GA.25
Data From Imaging Modalities
Fundus autofluorescence generated by short-wavelength exciting light is due mostly to lipofuscin.26 The emitted light is in the 500- to 750-nm range (peak ~630 nm). Most of the lipofuscin signal arises from the RPE, and loss of FAF is associated with loss of RPE.
Adaptive optics SLO data indicate, however, that the converse may not be true.27 Visual cycle inhibitors, eg, emixustat (Acucela, Inc., Seattle, WA) and fenretinide, are intended to reduce reactive oxygen species formation in RPE by reducing 11-cis-retinal formation, in turn reducing A2E formation.28,29
These compounds have been documented to reduce RPE FAF in preclinical models of Stargardt disease,30 and their ability to prevent GA expansion is under investigation.29,31
It may be relevant that a study of histologic autofluorescence in GA donor eyes revealed that areas with advanced RPE alterations were most likely to exhibit increased FAF around the margins GA, but these areas were associated with vertically superimposed cells and cell fragments.32 As a result, areas of increased FAF may not predict the locus of RPE cells that are about to die.
IMAGING MELANIN
As Spaide and Curcio33 pointed out, melanin (eumelanin) is a brown-black polymer of dihydroxyindole carboxylic acid and its reduced forms. Melanosomes, melanin-containing rod-shaped organelles, are present predominantly in the RPE apical cytoplasm and in the apical processes that envelop photoreceptor outer segment tips. RPE melanosomes absorb light from the blue end (short-wavelength) of the visible spectrum preferentially.
Melanin is the main source of NIA in a healthy fundus.34 Schmitz-Valckenberg et al,35 for example, compared confocal SLO in vivo imaging using blue vs near-infrared (excitation: 790 nm, emission: >810 nm) FAF in patients with GA, as well as in pigmented and albino rats. Histology in pigmented rats and in a human donor eye indicated that the NIA signal origin localized to the RPE and sclera. No NIA was detected in the retinas of young nonpigmented rats.
As in blue FAF, in NIA, retinal vessels and the optic disc appear dark. In contrast to blue FAF, in NIA, no macular carotenoid-associated decrease in autofluorescence occurs at the fovea. In the future, important information about early disease progression may be found in the preferential loss of melanin fluorescence vs lipofuscin fluorescence.
IMAGING FLUORESCEIN
Fluorescein, or resorcinolphthalein, is an organic compound that fluoresces (absorption maximum 494 nm, emission maximum 521 nm in water) when illuminated with short-wavelength light.
Fundus cameras typically are equipped with filters that limit incident (blue) light to 465-490 nm (exciter filter) and that allow fluorescent light only of longer (yellow-green) wavelength, typically 520-530 nm (barrier filter), to reach the fundus camera.
Fluorescein angiography can image drusen and the retinal vasculature, as well as pigment epithelial abnormalities (eg, RPE tears) and new choroidal vessels. Occasionally, the physician can see the choriocapillaris and larger choroidal vessels if the RPE is absent or is relatively depigmented. (Melanin in the RPE tends to absorb the exciting and emitted light on FA.)
IMAGING INDOCYANINE GREEN
Near-infrared fundus illumination is employed most often during ICG angiography. The subretinal and choroidal anatomy is more clearly delineated with long-wavelength light than with the shorter-wavelength light used in FA.36
Indocyanine green is a cyanine dye with peak absorption of 800 nm (range 600-900 nm) and peak fluorescence at 830 nm (range 750-950 nm). ICG is highly bound to plasma proteins. The longer wavelength of incident and fluorescent light (vs fluorescein) allows the physician to visualize structures in the choroid more easily with ICG angiography than with FA.
Indocyanine green angiography is used primarily to identify areas of CNV, but it also can localize areas of choroidal nonperfusion well. Piccolino et al37 also noted a high (~40%) incidence of preinjection fluorescence at 815 nm that is likely autofluorescence.
OPTICAL COHERENCE TOMOGRAPHY
OCT uses reflected light to create cross-sectional and three-dimensional images of objects. Time-domain OCT compares reflected light to light from a reference mirror (typically near infrared light, 800-1,310 nm).38
Time delays in the reflected light that result from absorption or dispersion by tissue are compared with the light from the reference mirror to determine the depth of the signal.
Spectral (or Fourier)-domain OCT uses a spectrophotometer to measure the difference in wavelength between the reference beam and light reflected from the tissue.39
The wavelength data are analyzed by Fourier transformation to create spatial data. In contrast to TD-OCT, there is no moving reference arm, so image acquisition is one to three orders of magnitude faster. SD-OCT also provides greater image resolution (3- to 5-µm axial resolution) than TD-OCT (8- to 10-µm axial resolution).
In addition, the increased scanning speed, registration software, and tracking technology enable the physician to establish point-to-point correlations between OCT scans and other imaging modalities, including FAF, NIA, NIR, FA, AOSLO, and color fundus photographs.
Beyond SD-OCT
Swept-source OCT uses a short cavity-swept laser (vs a superluminsecent diode used in SD-OCT) to achieve even higher acquisition rates at a longer wavelength (1,060 nm), compared with SD-OCT imaging.40
The higher acquisition rate and longer wavelength translate into higher-density scans, larger scan areas, better tissue penetration, and the ability to perform OCT angiography (phase-variance OCT) of the retinal and choroidal vasculature.
Conventional OCT imaging provides limited visualization of the retinal and choroidal vasculature. Enhanced depth imaging (EDI)-OCT, however, yields better visualization of the choroid than conventional OCT.41,42
Using OCT angiography makes it possible to obtain high-resolution images of the retinal and choroidal vasculature in patients, including those with AMD (Figure 3, page 33).43,44
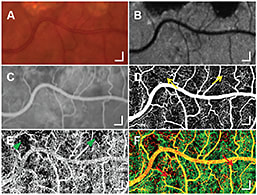
Figure 3. Identification of an abnormality in CC at the edges of GA for a scanning area of 3 × 1.5 mm2. Selected regions of a color fundus photograph (A), AF (B), and an early phase of FA (C). An en face image (D) from pvOCT data shows fine normal retinal capillaries (yellow arrows) at the edge of GA, which are not as well visualized with FA (C). A CC perfusion map (E) from pvOCT illustrates small focal areas of CC dropout near the inferior edge of GA, which correlates with the areas of irregular choroidal filling on FA (C). A color composite image (F) of retinal circulation (D, red) and CC (E, green) from pvOCT. (Scale bars, 200 μm.)
CREDIT: US NATIONAL ACADEMY OF SCIENCE
Possible Applications
This technology may allow us to correlate structural changes in the RPE and photoreceptors (visualized with SS-OCT) with vascular changes in the retina and choroid, using OCT technology that keeps all of the visualized structures in correct anatomic register.
In contrast to other OCT-based approaches to imaging the retinal and choroidal vasculature,45-47 phase-variance OCT, as Kim et al43 and Schwartz et al44 described, does not require hardware modification of the OCT machine or nonconventional scanning patterns.
In addition, increased scanning speeds will not compromise the ability of phase-variance OCT to provide high-quality images (vs some Doppler-based techniques48).
Obtaining phase-variance OCT images using the methods of Kim et al43 and Schwartz et al44 only requires analysis of the data (via specialized software) already acquired during routine SD-OCT scanning.
ADAPTIVE OPTICS
Adaptive optics SLO can produce images of individual cone photoreceptors in living patients, as well as images of the RPE mosaic. By compensating for ocular wavefront aberrations, adaptive optics provides lateral image resolution of 2 µm, which enables the physician to visualize individual cone photoreceptors reproducibly and longitudinally.49-51
A typical AOSLO system uses low-coherence, long-wavelength incident light (eg, 840 nm), a Shack-Hartmann wavefront sensor, and a microelectromechanical systems-manufactured deformable mirror to correct the measured wavefront abnormalities.52
The wavefront sensor measures ocular aberrations and corrects for them using a wavefront corrector to project a corrected wavefront onto an imaging device (eg, a fundus camera).
Some specific challenges arise when using AOSLO in AMD patients due to the frequent presence of cataract, dry eye, and poor fixation (due to poor vision), which result in increased image scattering, increased distortion in scanning system images, and compromised image registration.27
NEAR-INFRARED FUNDUS REFLECTANCE
Confocal near-infrared (830 nm) fundus reflectance is a noninvasive en face imaging technique.36 As noted above, subretinal features are more easily visualized with near-infrared light than with visible light.36
Subretinal deposits appear light and thickened, whereas the optic nerve head, retinal vessels, and choroidal vessels appear dark (Figure 4, page 34). The contrast and visibility of features increases with wavelength from 795 to 895 nm.36 Weinberger et al34 observed a strong correlation between NIA and increased NIR at identical fundus locations. Melanin is the most likely candidate for the cause of NIA and NIR.34 Areas of GA appear as bright regions on NIR.53
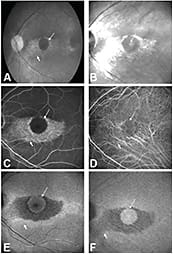
Figure 4. Chloroquine maculopathy. The arrow points to a circular area of condensed pigment; the arrowhead an area of pigment atrophy. A) Fundus photograph. B) NIR reflectance increases in the area of depigmentation (arrowhead). C) Fluorescein angiogram. D) ICG angiogram. E) Blue-light–excited autofluorescence showing almost regular blue-light–excited autofluorescence in the circular area of condensed pigment, whereas the rim of the atrophy shows increased blue-light–excited autofluorescence (arrowhead). F) The NIR fluorescence signal is strongest in the central circular area. The increased NIR reflectance does not contribute to NIR fluorescence (arrowhead).
CREDIT: ASSN FOR RESEARCH IN VISION AND OPHTHALMOLOGY
Fibrin also appears bright on NIR.54 Confocal laser ophthalmoscopes provide higher-quality NIR images than camera-based systems, due to the ability of confocal systems to separate reflected and scattered light.
LOOKING AHEAD
In the second part of this review, we will discuss the application of these new imaging modalities in dry AMD. These emerging technologies could provide fresh opportunities to diagnose and treat GA. RP
Editor’s note: This article will appear in slightly altered form in a forthcoming issue of Developmental Ophthalmology, to be published later this year by S. Karger AG (Basel, Switzerland) and on which Dr. Zarbin is a coeditor. Retinal Physician acknowledges S. Karger for the opportunity to publish this research.
REFERENCES
1. AREDS2-HOME Study Research Group; Chew WC, Clemons TE, Bressler SB, et al. Randomized trial of a home monitoring system for early detection of choroidal neovascularization Home Monitoring of the Eye (HOME) study. Ophthalmology. 2014;121:535-544.
2. Zarbin MA, Casaroli-Marano RP, Rosenfeld PJ. Age-related macular degeneration: clinical findings, histopathology, imaging techniques. In: Casaroli-Marano RP, Zarbin, MA, eds. Cell-Based Therapy for Retinal Degenerative Disease. Basel, Switzerland: Karger Medical and Scientific Publishers; in press:1-32.
3. Ferris FL, Davis MD, Clemons TE, et al. A simplified severity scale for age-related macular degeneration: AREDS Report No. 18. Arch Ophthalmol. 2005;123:1570-1574.
4. Wang JJ, Foran S, Smith W, Mitchell P. Risk of age-related macular degeneration in eyes with macular drusen or hyperpigmentation: the Blue Mountains Eye Study cohort. Arch Ophthalmol. 2003;121:658-663.
5. Klein R, Klein BE, Tomany SC, Meuer SM, Huang GH. Ten-year incidence and progression of age-related maculopathy: The Beaver Dam eye study. Ophthalmology. 2002;109:1767-1779.
6. van Leeuwen R, Klaver CC, Vingerling JR, Hofman A, de Jong PT. The risk and natural course of age-related maculopathy: follow-up at 6 1/2 years in the Rotterdam study. Arch Ophthalmol. 2003;121:519-526.
7. Age-Related Eye Disease Study Research Group. The Age-Related Eye Disease Study system for classifying age-related macular degeneration from stereoscopic color fundus photographs: the Age-Related Eye Disease Study Report Number 6. Am J Ophthalmol. 2001;132:668-681.
8. Jain N, Farsiu S, Khanifar AA, et al. Quantitative comparison of drusen segmented on SD-OCT versus drusen delineated on color fundus photographs. Invest Ophthalmol Vis Sci. 2010;51:4875-4883.
9. Leuschen JN, Schuman SG, Winter KP, et al. Spectral-domain optical coherence tomography characteristics of intermediate age-related macular degeneration. Ophthalmology. 2013;120:140-150.
10. Folgar FA, Chow JH, Farsiu S, et al. Spatial correlation between hyperpigmentary changes on color fundus photography and hyperreflective foci on SDOCT in intermediate AMD. Invest Ophthalmol Vis Sci. 2012;53:4626-4633.
11. Zarbin MA. Current concepts in the pathogenesis of age-related macular degeneration. Arch Ophthalmol. 2004;122:598-614.
12. Jin GF, Hurst JS, Godley BF. Rod outer segments mediate mitochondrial DNA damage and apoptosis in human retinal pigment epithelium. Curr Eye Res. 2001;23:11-19.
13. Jarrett SG, Lin H, Godley BF, Boulton ME. Mitochondrial DNA damage and its potential role in retinal degeneration. Prog Retin Eye Res. 2008;27:596-607.
14. Liang FQ, Godley BF. Oxidative stress-induced mitochondrial DNA damage in human retinal pigment epithelial cells: a possible mechanism for RPE aging and age-related macular degeneration. Exp Eye Res. 2003;76:397-403.
15. Feher J, Kovacs I, Artico M, Cavallotti C, Papale A, Balacco Gabrieli C. Mitochondrial alterations of retinal pigment epithelium in age-related macular degeneration. Neurobiol Aging. 2006;27:983-993.
16. Jones MM, Manwaring N, Wang JJ, Rochtchina E, Mitchell P, Sue CM. Mitochondrial DNA haplogroups and age-related maculopathy. Arch Ophthalmol. 2007;125:1235-1240.
17. Kanda A, Chen W, Othman M, et al. A variant of mitochondrial protein LOC387715/ARMS2, not HTRA1, is strongly associated with age-related macular degeneration. Proc Natl Acad Sci U S A. 2007;104:16227-16232.
18. Vives-Bauza C, Anand M, Shirazi AK, et al. The age lipid A2E and mitochondrial dysfunction synergistically impair phagocytosis by retinal pigment epithelial cells. J Biol Chem. 2008;283:24770-24780.
19. Berg JM, Tymoczko JL, Stryer L. Biochemistry. 6th ed. New York, NY; W.H. Freeman; 2007.
20. Weber G. Fluorescence of riboflavin and flavin-adenine dinucleotide. Biochem J. 1950;47:114-121.
21. Reinert KC, Dunbar RL, Gao W, Chen G, Ebner TJ. Flavoprotein autofluorescence imaging of neuronal activation in the cerebellar cortex in vivo. J Neurophysiol. 2004;92:199-211.
22. Elner SG, Elner VM, Field MG, Park S, Heckenlively JR, Petty HR. Retinal flavoprotein autofluorescence as a measure of retinal health. Trans Am Ophthalmol Soc. 2008;106:215-222; discussion 222-214.
23. Kennedy CJ, Rakoczy PE, Constable IJ. Lipofuscin of the retinal pigment epithelium: a review. Eye. 1995;9:763-771.
24. Sparrow JR, Fishkin N, Zhou J, et al. A2E, a byproduct of the visual cycle. Vision Res. 2003;43:2983-2990.
25. Holz FG, Bindewald-Wittich A, Fleckenstein M, Dreyhaupt J, Scholl HP, Schmitz-Valckenberg S. Progression of geographic atrophy and impact of fundus autofluorescence patterns in age-related macular degeneration. Am J Ophthalmol. 2007;143:463-472.
26. Delori FC, Dorey CK, Staurenghi G, Arend O, Goger DG, Weiter JJ. In vivo fluorescence of the ocular fundus exhibits retinal pigment epithelium lipofuscin characteristics. Invest Ophthalmol Vis Sci. 1995;36:718-729.
27. Rossi EA, Rangel-Fonseca P, Parkins K, et al. In vivo imaging of retinal pigment epithelium cells in age related macular degeneration. Biomed Opt Express 2013;4:2527-2539.
28. Zarbin MA, Rosenfeld PJ. Pathway-based therapies for age-related macular degeneration: an integrated survey of emerging treatment alternatives. Retina. 2010;30:1350-1367.
29. Kubota R, Boman NL, David R, Mallikaarjun S, Patil S, Birch D. Safety and effect on rod function of acu-4429, a novel small-molecule visual cycle modulator. Retina. 2012;32:183-188.
30. Radu RA, Han Y, Bui TV, et al. Reductions in serum vitamin A arrest accumulation of toxic retinal fluorophores: a potential therapy for treatment of lipofuscin-based retinal diseases. Invest Ophthalmol Vis Sci. 2005;46:4393-4401.
31. Mata NL, Lichter JB, Vogel R, Han Y, Bui TV, Singerman LJ. Investigation of oral fenretinide for treatment of geographic atrophy in age-related macular degeneration. Retina. 2013;33:498-507.
32. Rudolf M, Vogt SD, Curcio CA, et al. Histologic basis of variations in retinal pigment epithelium autofluorescence in eyes with geographic atrophy. Ophthalmology. 2013;120:821-828.
33. Spaide RF, Curcio CA. Drusen characterization with multimodal imaging. Retina. 2010;30:1441-1454.
34. Weinberger AW, Lappas A, Kirschkamp T, et al. Fundus near infrared fluorescence correlates with fundus near infrared reflectance. Invest Ophthalmol Vis Sci. 2006;47:3098-3108.
35. Schmitz-Valckenberg S, Lara D, Nizari S, et al. Localisation and significance of in vivo near-infrared autofluorescent signal in retinal imaging. Br J Ophthalmol. 2011;95:1134-1139.
36. Elsner AE, Burns SA, Weiter JJ, Delori FC. Infrared imaging of sub-retinal structures in the human ocular fundus. Vis Res. 1996;36:191-205.
37. Piccolino FC, Borgia L, Zinicola E, Iester M, Torrielli S. Pre-injection fluorescence in indocyanine green angiography. Ophthalmology. 1996;103:1837-1845.
38. Huang D, Swanson EA, Lin CP, et al. Optical coherence tomography. Science. 1991;254:1178-1181.
39. Costa RA, Skaf M, Melo LA, Jr., et al. Retinal assessment using optical coherence tomography. Prog Retin Eye Res. 2006;25:325-353.
40. Potsaid B, Baumann B, Huang D, et al. Ultrahigh speed 1050nm swept source/Fourier domain OCT retinal and anterior segment imaging at 100,000 to 400,000 axial scans per second. Opt Express. 2010;18:20029-20048.
41. Spaide RF, Koizumi H, Pozzoni MC. Enhanced depth imaging spectral-domain optical coherence tomography. Am J Ophthalmol. 2008;146:496-500.
42. Imamura Y, Fujiwara T, Margolis R, Spaide RF. Enhanced depth imaging optical coherence tomography of the choroid in central serous chorioretinopathy. Retina. 2009;29:1469-1473.
43. Kim DY, Fingler J, Zawadzki RJ, et al. Optical imaging of the chorioretinal vasculature in the living human eye. Proc Natl Acad Sci U S A. 2013;110:14354-14359.
44. Schwartz DM, Fingler J, Kim DY, et al. Phase-variance optical coherence tomography. Ophthalmology. 2014;121:180-187.
45. Zotter S, Pircher M, Torzicky T, et al. Visualization of microvasculature by dual-beam phase-resolved Doppler optical coherence tomography. Opt Express. 2011;19:1217-1227.
46. Makita S, Jaillon F, Yamanari M, Miura M, Yasuno Y. Comprehensive in vivo micro-vascular imaging of the human eye by dual-beam-scan Doppler optical coherence angiography. Opt Express. 2011;19:1271-1283.
47. Braaf B, Vermeer KA, Vienola KV, de Boer JF. Angiography of the retina and the choroid with phase-resolved OCT using interval-optimized backstitched B-scans. Opt Express. 2012;20:20516-20534.
48. Grulkowski I, Gorczynska I, Szkulmowski M, et al. Scanning protocols dedicated to smart velocity ranging in spectral OCT. Opt Express. 2009;17:23736-23754.
49. Liang J, Williams DR, Miller DT. Supernormal vision and high-resolution retinal imaging through adaptive optics. J Opt Soc Am A Opt Image Sci Vis. 1997;14:2884-2892.
50. Roorda A, Romero-Borja F, Donnelly Iii W, Queener H, Hebert T, Campbell M. Adaptive optics scanning laser ophthalmoscopy. Opt Express. 2002;10:405-412.
51. Talcott KE, Ratnam K, Sundquist SM, et al. Longitudinal study of cone photoreceptors during retinal degeneration and in response to ciliary neurotrophic factor treatment. Invest Ophthalmol Vis Sci. 2011;52:2219-2226.
52. Zhang Y, Poonja S, Roorda A. MEMS-based adaptive optics scanning laser ophthalmoscopy. Opt Lett. 2006;31:1268-1270.
53. Fazelat A, Desai M, Nandakumar N, Lowry NC, Mangoubi R, Lashkari K. Enhanced, near-infrared fundus reflectance for qualitative and quantitative analysis of subretinal lesions. Exp Eye Res. 2012;96:171-177.
54. Patton N, Aslam TM, MacGillivray T, et al. Retinal image analysis: concepts, applications and potential. Prog Retin Eye Res. 2006;25:99-127.
55. Sarks S. Ageing and degeneration in the macular region: a clinico-pathological study. Br J Ophthalmol. 1976;60:324-341.
56. Green WR, Enger C. Age-related macular degeneration histopathologic studies. The 1992 Lorenz E. Zimmerman Lecture. Ophthalmology. 1993;100:1519-1535.
57. Hageman GS, Luthert PJ, Victor Chong NH, Johnson LV, Anderson DH, Mullins RF. An integrated hypothesis that considers drusen as biomarkers of immune-mediated processes at the RPE-Bruch’s membrane interface in aging and age-related macular degeneration. Prog Retin Eye Res. 2001;20:705-732.
58. Karwatowski WS, Jeffries TE, Duance VC, Albon J, Bailey AJ, Easty DL. Preparation of Bruch’s membrane and analysis of the age-related changes in the structural collagens. Br J Ophthalmol. 1995;79:944-952.
59. Nozaki M, Raisler BJ, Sakurai E, et al. Drusen complement components C3a and C5a promote choroidal neovascularization. Proc Natl Acad Sci U S A. 2006;103:2328-2333.
60. Starita C, Hussain AA, Pagliarini S, Marshall J. Hydrodynamics of ageing Bruch’s membrane: implications for macular disease. Exp Eye Res. 1996;62:565-572.
61. Haimovici R, Gantz DL, Rumelt S, Freddo TF, Small DM. The lipid composition of drusen, Bruch’s membrane, and sclera by hot stage polarizing light fmicroscopy. Invest Ophthalmol Vis Sci. 2001;42:1592-1599.
62. Marshall J, Hussain AA, Starita C, Moore DJ, Patmore AL. Aging and Bruch’s membrane. In: Marmor MF, Wolfensberger TJ, eds. The Retinal Pigment Epithelium. New York, NY; Oxford University Press; 1998:669-692.
63. Curcio CA, Johnson M, Huang JD, Rudolf M. Apolipoprotein B-containing lipoproteins in retinal aging and age-related macular degeneration. J Lipid Res. 2010;51:451-467.
64. Handa JT, Verzijl N, Matsunaga H, et al. Increase in the advanced glycation end product pentosidine in Bruch’s membrane with age. Invest Ophthalmol Vis Sci. 1999;40:775-779.