What Is the Role of Ischemia in AMD?
Early detection of ischemic damage may allow for the diagnosis of progression before changes manifest clinically.
Shani Golan, MD • Dov Weinberger, MD • Tami Livnat, PhD • Anat Loewenstein, MD
Age-related macular degeneration is the leading cause of legal blindness among older individuals in the developed world, occurring primarily in people aged 50 and older.1-3 The Blue Mountain study group identified the 10-year incidences of early AMD and late AMD to be 10.8% and 2.8%, respectively.4 The Cardiovascular Health and Age-related Maculopathy study demonstrated that the risk of early AMD progression was between 3.4% and 4.7% per year and the risk of late AMD progression was 0.9% per year.5
It has been predicted that as the population ages, there will be a 50% increase in the incidence of AMD before 2020.6 In another study, AMD was reported to account for 54% of the cases of blindness among the Caucasian population in the United States, and the number of blind people could increase by as much as 70% by 2020.7
This review provides a brief summary of AMD's proposed pathogenesis, with an emphasis on ischemia. Supporting evidence for this theory is provided by imaging studies using the Retinal Function Imager (RFI).
REVIEW OF DEFINITIONS
As retinal specialists are well aware, AMD affects the retinal pigment epithelium and leads to a progressive photoreceptor degeneration in the central retina, with associated loss of central vision.8-9 AMD can be divided into early, intermediate and late forms.10-11
Shani Golan, MD, is on the faculty of the Department of Ophthalmology, Tel Aviv Medical Center Sackler Faculty of Medicine, Tel Aviv University, in Tel Aviv, Israel. Dov Weinberger, MD, and Tami Livnat, PhD, are on the faculty of the Department of Ophthalmology, Rabin Medical Center, Laboratory of Eye Research, Felsenstein Medical Research Center, in Petah Tikva, Israel, and on the faculty at Sackler. Anat Loewenstein, MD, is on the faculty at Sackler. None of the authors reports any financial interest in any products mentioned in this article. Dr. Loewenstein can be reached via e-mail at anatl@tasmc.health.gov.il. |
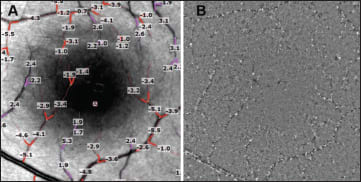
Figure 1. Quantification of retinal blood-flow velocity in the human retina. A. RFI image showing calculated velocity in mm/sec, obtained automatically. The image shows several arteries (reddish) and veins (pink) that were manually selected for quantification. A negative value indicates blood flow away from the heart, whereas a positive value indicates blood flow toward the heart. B. Single frame of a flow movie from the same human retina. Erythrocyte clusters (black) and gaps (white) move through the small vessels shown here at an average velocity rate of 2 to 3 mm/sec.
Drusen, the hallmark of early and intermediate AMD, can be found in about 85% of patients.10 These amorphous deposits accumulate between the RPE and the inner collagenous zone of Bruch's membrane. Ophthalmoscopically, drusen can be divided into hard (less than 63 µm) and soft (greater than 125 µm) drusen. Soft drusen are a major risk factor for developing more advanced stages of AMD, particularly when they are accompanied by pigment irregularities, such as hyper- and hypopigmentation.11
Analysis has revealed many protein remnants and a number of inflammatory and immune-associated elements.12-13
Intermediate AMD is characterized by the accumulation of more than six focal or diffuse drusen measuring greater than 125 µm and hyper- or hypopigmentation of the RPE.
Advanced AMD can be classified into either of two categories. The first of these comprises the geographic atrophy typical of nonexudative or “dry” AMD, which is characterized by a sharply delineated area of RPE atrophy measuring at least 175 µm along one dimension and including visible choroidal vessels.10 Alternatively, there is the neovascular or “wet” form of AMD, in which there is new vessel growth through Bruch's membrane from the underlying choroid. Wet AMD may involve some or all of the following: subretinal neovascular membranes, subretinal fluid, exudates, and hemorrhages. It causes acute central vision loss in about 15% of AMD patients.
RISK FACTORS
Although advancing age is the greatest risk factor associated with the development of AMD, environmental and lifestyle factors may significantly affect individual risk. Smoking is an important, modifiable factor. The risk of macular degeneration is two- to threefold higher in current smokers compared with individuals who have never smoked.14 Oxidative stress and antioxidant depletion have been implicated in retinal damage from smoking, although the precise mechanism remains unclear.15,16 Other factors that have been reported to influence risk for AMD include sunlight exposure, alcohol consumption, cardiovascular disease, history of diabetes, increased plasma fibrinogen levels, diet, hypertension, body mass index and iris color.11,17-19 Regarding diet, antioxidants, such as carotenoids, zinc, and vitamins A and E, may provide a protective benefit against AMD.
The condition is also thought to be more prevalent among Caucasians, compared with either African Americans or people of Hispanic origin.20 Such ethnic differences may reflect genetic, as well as environmental, risk factors.
AMD has a significant genetic component,21-22 as several twin studies have shown significantly higher concordance rates between monozygotic twins as compared to concordance between dizygotic twins.23 Family members of individuals with AMD are at increased risk (2.4- to 19.8- fold) for developing the disease relative to individuals with no family history.24
PATHOGENESIS
The etiology and pathophysiology of AMD are poorly understood.
Drusen are the histological markers of AMD. The cause of drusen may be linked to one or more of the following key processes that are implicated in AMD.
(1) Increased outer segment turnover.
(2) Free radical/oxidative damage.
(3) Aging and degeneration of elements of Bruch's membrane (eg, collagen and elastin).
(4) Reduced clearance of material from Bruch's membrane into the choriocapillaries. Specific alterations in Bruch's membrane composition that have been suggested to cause drusen include excessive lipid deposition and protein cross-linking as well as impaired permeability to nutrients.
(5) Deleterious immune system activation.25
(6) Impaired activity/function of the RPE. Several changes in the RPE structure contribute to the development of AMD; among them are structural changes that occur in the tight-belted monolayer of the RPE and disrupt the outer blood retina barrier (oBRB). These changes contribute to the cascade of AMD development, especially the wet form of the disease.
These processes are triggered by several mechanisms; inflammation and ischemia are among the major pathologic mechanisms.
AMD AND THE DISRUPTION OF THE oBRB
The RPE is the major component of the oBRB, separating the neural retina from a network of fenestrated choriocapillaris at the innermost vascular layer of the choroid. This layer lies adjacent to Bruch's membrane and is composed of small but richly anastomotic capillaries. The choroidal vasculature layer is the main supporter of the metabolic and oxygen requirements of the RPE cells and photoreceptors.26
The oBRB regulates the composition of the subretinal space, enabling the photoreceptors to carry out their functions. The RPE plays many essential roles including transport of nutrients, water and ions and the removal of metabolic waste. It is responsible for daily phagocytosis and renewal of the outer segment discs of the photoreceptors and plays a crucial role in the transport and recycle of fatty acids. It is also responsible for the removal of excessive water from the subretinal space toward the choriocapillaris.27 AMD is often diagnosed by the appearance of subretinal fluid. This fluid creates a local detachment of the retina in the macular area resulting in decreased visual acuity.28
The oBRB integrity is maintained by a series of tight junction (TJ) proteins, responsible for regulating RPE cell-to-cell contact and limiting the intercellular space, thus controlling the passive paracellular transport and enabling the active transport of needed materials. The TJ family includes more than 40 proteins, among them trans-membrane proteins, folding proteins and signal transduction proteins.29 The TJ system is a dynamic system capable of regulating the intercellular space and controlling the passage of substances of varying size. The TJ belt divides the RPE membrane into its basal and epical sides, creating polarity in the RPE monolayer and moving its different functional needs in the direction of either the photoreceptors or the choroid.2
Various studies have connected the functional changes in TJ proteins of the RPE to the pathophysiology of AMD.30,31 In addition, vascular endothelial growth factor can cause rapid post-translational modifications of TJ proteins by stimulating occludin phosphorylation and by inducing tyrosine phosphorylation of ZO-1.31 Increased intraocular VEGF production occurs in ischemic retinal diseases as well as choroidal vascular diseases, and it has a direct influence on the oBRB structure.
Disruption of the integrity of the RPE barrier also occurs when a neovascular membrane penetrates the RPE monolayer to invade the subretinal space, as found in the neovascular form of AMD. A recent work32 demonstrated that direct pathological contact between RPE and endothelial cells (which are normally separated) enhances the proangiogenic potential of the endothelial cells to proliferate and migrate, similar to the process induced by hypoxia. These findings support the linkage between pathological changes in the RPE and the development of angiogenesis involved in CNV formation.
ISCHEMIA AND HYPOXIA IN AMD DEVELOPMENT
Considering ischemia and hypoxia as key factors in the pathophysiology of AMD, these terms should be clarified. Choroidal ischemia is determined as insufficient blood supply to the retina. In ischemia, the partial pressure of oxygen within the tissue is reduced due to inadequate oxygen supply to the retina. Furthermore, hypoxia means reduced oxygen levels within the cells, leading to reductions in mitochondrial respiration and oxidative metabolism. A prolonged hypoxic state may lead to cell death. Ischemia always results in hypoxia; however, cellular hypoxia can occur without ischemia even in an environment rich in oxygen.
In the normal eye, oxygen diffuses from the choriocapillaris through Bruch's membrane and RPE towards the avascular outer retina and is consumed mainly by the photoreceptors. The level of oxygen in the RPE is usually very high because of the close proximity between the RPE and the choriocapillaris. The level of oxygen decreases almost linearly as the distance from the choriocapillaris to the inner portion of the photoreceptors increases.
Pathological changes, such as drusen accumulation, thickening of Bruch's membrane, water accumulation, pigment epithelial detachment and retinal detachment, increase the distance between the choriocapillaris and the retina,33-35 reduce the oxygen flux to the photoreceptors and induce hypoxia in the retinal cells. Low oxygen levels in the cellular environment induce elevation in hypoxia-inducible factor, a transcription factor that further activates VEGF gene expression, thus leading to increases in permeability and angiogenesis.
The theory that choroidal and retinal blood flow abnormalities play a role in AMD is also well established. Vascular deficits due to reduced choroidal and retinal blood flow have been identified in early and late AMD using fluorescein angiography, Doppler flowmetry and Doppler imaging.36-40 Reduced blood flow in the choroid and retina causes chronic ischemia in Bruch's membrane, the RPE and the neuroretina.36,41 Both drusen and RPE abnormalities are associated with decreased choroidal blood flow.36 Impaired perfusion might cause impaired RPE function that then results in decreased degradation of photoreceptor disc membranes, reduced antioxidant capacity and an increased number of drusen in Bruch's membrane.
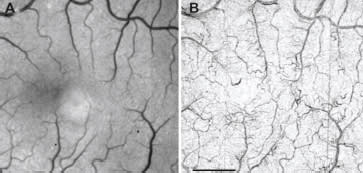
Figure 2. Invisible vasculature (in red-free images) revealed noninvasively by the RFI. A. Red-free image of a healthy subject. B. The RFI's capillary perfusion map, showing fine microvasculature detail (scale bar = 1 mm).
Compromised RPE cell function causes a diminution of vascular growth factors such as vascular endothelial growth factor from the choroid. VEGF depletion induces choroidal atrophy42 and further delays perfusion. The thickening of Bruch's membrane due to drusen not only leads to reduced diffusion of oxygen and thus pronounced neuroretinal ischemia but also to impaired transport capacity of important metabolites of the retinoid cycle (such as vitamin A).43
Feigl et al. proposed the ischemia postreceptoral hypothesis.41 They suggested that photoreceptors are more resistant to ischemic insult than postreceptoral neurons, owing to their proximity to metabolic reserves available in the RPE and the choriocapillaris. The postreceptoral neurons are particularly vulnerable, as they are located in the watershed zone between two blood supplies,41 the choroidal and retinal vascular systems. This hypothesis is supported by histopathogical findings. When the RPE degenerates, the postreceptoral outer plexiform and inner nuclear layers are first affected.44 It is still not clear which retinal layer is first targeted by ischemia in humans; whether it is the outer45 or the inner retina46,47 needs to be confirmed.
RETINAL FUNCTION IMAGER
In recent years, several attempts have been made to develop new retinal imaging technologies.48-51 However, most systems available for clinical use monitor structural changes in the retina and do not provide direct information about the retina's functioning. The Retinal Function Imager (RFI) is a digital imaging system designed to capture accurate retinal images via a standard fundus camera. It offers a noninvasive diagnostic approach to retinal function assessment and provides easy, direct, qualitative and quantitative imaging of four parameters: (1) blood-flow velocity,52-54 (2) capillary perfusion maps,52-54 (3) blood oximetry 52-54 and (4) metabolic intrinsic state.52-54 All of these parameters directly assess retinal function and are thought to be modified in the course of retinal diseases. Most importantly, as retinal functional changes precede structural changes, quantitative imaging of these four parameters provides a useful tool for early detection of retinal diseases in general and AMD in particular.53,54
The four key parameters offered by the new imager are:
1. Blood flow. Under green illumination, hemoglobin within the erythrocytes in the bloodstream provides a natural, high-contrast chromophore for tracking blood flow. Cross-correlation matching of moving erythrocyte patterns within an image series gives a direct measure of velocity. Reregistration and differential processing of a series of images taken at 55 Hz produces a “flow movie,” in which it is possible to follow the motion of individual clusters of red blood cells — or even single red blood cells — in vessels as small as capillaries. Flow movies provide a tool for qualitative assessment of retinal blood-flow rates.
In a study of AMD patients, the RFI detected reduced blood-flow velocity in exudative AMD eyes compared with fellow dry AMD eyes (in all sizes of veins and in small arteries; mean age, 78.5 ± 4.5 years).55 Drug effects on the retina have also been studied with the RFI. Following intravitreal bevacizumab injections, a distinctive pattern of change in patient retinal blood-flow velocity was noted in responders vs nonresponders.55
A recent study assessed the short-term effects of intravitreal bevacizumab on retinal blood-flow velocity compared to clinical outcomes assessed by optical coherence tomography and tests of visual acuity.56 Eight patients receiving intravitreal injection of bevacizumab for CNV were included. All were imaged by the RFI preinjection and at one and seven days postinjection. VA and OCT were recorded preinjection and at one month postinjection. The researchers found a good correlation between the one-month change in VA and OCT measurements and the short-term change induced in bloodflow velocity. Arterial and venous velocity changes one day after the injection correlated with the VA change (P<.05). The one-day arterial velocity changes correlated with total macular volume (P=.02) and venous velocity changes correlated with central macular thickness (P=.04).
In a different study, Landa et al.57 compared venous blood-flow velocity with central retinal volume in patients with various ocular diseases and observed a strong correlation between venous retinal blood-flow velocity and central retinal volume. The RFI may thus be a useful tool for evaluating changes in retinal thickening.
2. Capillary perfusion maps. The ability to directly witness the motion of red blood cell aggregates provides a tool to map the surface retinal vasculature in detail. Following image registration, pixel value distribution parameters are analyzed to locate blood motion, tracing microvasculature based on motion contrast rather than on total reflectance contrast. The RFI's noninvasive capillary perfusion map (nCPM) shows vessels that are indistinct or invisible on even sharp red-free images. Indeed, small vessels often appear as clearly as larger ones on these maps because the red cell clusters moving in these vessels are more distinct and less motion blurring occurs.52 Recently, in studies with an improved algorithm,55 as much and often more microvascular detail could be seen on the nCPM than on corresponding fluorescein angiography images. The RFI can assist in cases where adverse reactions to fluorescein injection have been observed.58
3. Oximetry. The balance of oxygen supply and demand in the retina is closely regulated to maintain the processes of visual perception. Alterations in either oxygen supply or consumption might directly indicate the early onset of retinal abnormalities.59-60 Thus, it is important to have a tool for qualitative and quantitative assessment of oxygen utilization in the tissue. The difference between the absorption spectra of oxyhemoglobin and deoxyhemoglobin can be used to determine the oxygenation of blood with multispectral imaging methods. In multispectral imaging mode, the RFI can perform spectroscopic decomposition to qualitatively assess the oximetric state of the retina.
4. Metabolic function. The RFI is capable of imaging outside the absorption range of photoreceptors under near-infrared light (750 – 840 nm), and can be used to optically monitor retinal activity in response to a well-defined visual stimulus (562 ± 20 nm). The difference between the post-stimulated and prestimulated images is used to determine the metabolic state of the retinal compartments.
CONCLUSION
AMD is a multifactorial disease, and ischemic damage plays a major role in its development and progression.
Detection of functional parameter abnormalities may permit diagnosis of disease progression before anatomic abnormalities become evident, allowing treatment intervention before irreversible retinal damage occurs. The ongoing advances in the treatment of AMD, in conjunction with better diagnostic tools, will improve outcomes for patients suffering from this devastating disease. Furthermore, treatment can be improved by noninvasive follow-up.
The RFI's multimodal approach to functional imaging also opens research and drug development opportunities with regard to a wide range of retinal diseases, beyond the capabilities of structural imaging. RP
REFERENCES
1. Klein R, Klein BE, Linton KL. Prevalence of age-related maculopathy. The Beaver Dam eye study. Ophthalmology. 1992; 99:933-943.
2. Vingerling JR, Dielemans I, Hofman A, et al. The prevalence of age-related maculopathy in the Rotterdam Study. Ophthalmology. 1995; 102:205-210.
3. Hageman GS, Luthert PJ, Victor Chong NH, Johnson LV, Anderson DH, Mullins RF. An integrated hypothesis that considers drusen as biomarkers of immune-mediated processes at the RPE-Bruch's membrane interface in aging and age-related macular degeneration. Prog Retin Eye Res. 2001; 20:705-732.
4. Wang J, Rochtchina E, Lee A, et al. Ten year incidence and progression of age related maculopathy:the Blue Mountains Eye Study. Ophthalmology. 2007; 114:92-98.
5. Tikellis G, Robman L, Dimitrov P, Nicolas C, McCarty C, Guymer R. Characteristics of progression of early age-related macular degeneration:the Cardiovascular Health and Age-related Maculopathy Study. Eye. 2007; 21:169-176.
6. Friedman DS, O'Colmain BJ, Muñoz B. Prevalence of Age-Related Macular Degeneration in the United States. Arch Ophthalmol. 2004; 122:564-572.
7. Congdon N, O'Colmain B, Klaver CC. Causes and prevalence of visual impairment among adults in the United States. Arch Ophthalmol. 2004; 122:477-485.
8. Haddad S, Chen CA, Santangelo SL, Seddon JM. The genetics of age-related macular degeneration:a review of progress to date. Surv Ophthalmol. 2006; 51:316-363.
9. Rattner A, Nathans J. Macular degeneration:recent advances and therapeutic opportunities. Nat Rev Neurosci. 2006; 7:860-872.
10. Bird A, Bressler N, Bressler S, et al. An international classification and grading system for age-related maculopathy and age-related macular degeneration. The International ARM Epidemiological Study Group. Surv Ophthalmol. 1995; 39:367-374.
11. Age-Related Eye Disease Study Research Group. The Age-Related Eye Disease Study system for classifying age-related macular degeneration from stereoscopic color fundus photographs:the Age-Related Eye Disease Study Report Number 6. Am J Ophthalmol. 2001; 132:668-681.
12. Sarks S, Killingsworth M, Sarks J. Early drusen formation in the normal and aging eye and their relation to age related maculopathy:a clinicopathological study. Br J Ophthalmol. 1999; 83:358-368.
13. Hageman G, Luthert P, Victor Chong N, Johnson L, Anderson D, Mullins R. An integrated hypothesis that considers drusen as biomarkers of immunemediated processes at the RPE-Bruch's membrane interface in aging and age-related macular degeneration. Prog Retinal Eye Res. 2001; 20:705-732.
14. Thornton J, Edwards R, Mitchell P, et al. Smoking and age-related macular degeneration:a review of association. Eye. 2005; 19:935-944.
15. Dhubhghaill SS, Cahill MT, Campbell M, Cassidy L, Humphries MM, Humphries P. The pathophysiology of cigarette smoking and age related macular degeneration. Adv Exp Med Biol. 2010; 664:437-446.
16. Cano M, Thimmalappula R, Fujihara M, et al. Cigarette smoking, oxidative stress, the anti-oxidant response through Nrf2 signaling, and age-related macular degeneration. Vision Res. 2010; 50:652-664.
17. Hirvela H, Luukinen H, Laara E, Sc L, Laatikainen L. Risk factors of age-related maculopathy in a population 70 years of age or older. Ophthalmology. 1995; 103:871-877.
18. Seddon JM, Rosner B, Sperduto RD, et al. Dietary fat and risk for advanced age-related macular degeneration. Arch Ophthalmol. 2001; 119:1191-1199.
19. Montezuma SR, Sobrin L, Seddon JM. Review of genetics in age related macular degeneration. Semin Ophthalmol. 2007; 22:229-240.
20. Bressler SB, Munoz B, Solomon SD, West SK. Racial differences in the prevalence of age-related macular degeneration:the Salisbury Eye Evaluation (SEE) Project. Arch Ophthalmol. 2008; 126:241-245.
21. Heiba IM, Elston RC, Klein BE, Klein R. Sibling correlations and segregation analysis of age-related maculopathy: the Beaver Dam eye study. Genet Epidemiol. 1994; 11:51-67.
22. Klaver CC, Wolfs RC, Assink JJ, van Duijn CM, Hofman A, de Jong PT. Genetic risk of age-related maculopathy. Population-based familial aggregation study. Arch Ophthalmol. 1998; 116:1646-1651.
23. Grizzard SW, Arnett D, Haag SL. Twin study of age-related macular degeneration. Ophthalmic Epidemiol. 2003; 10:315-322.
24. Klein BE, Klein R, Lee KE, Moore EL, Danforth L. Risk of incident age-related eye diseases in people with an affected sibling: The Beaver Dam Eye Study. Am J Epidemiol. 2001; 154:207-211.
25. Gehrs KM, Anderson DH, Johnson LV, Hageman GS. Age-related macular degeneration—emerging pathogenetic and therapeutic concepts. Ann Med. 2006; 38:450-471.
26. Bill A. Blood circulation and fluid dynamics in the eye. Physiol Rev. 1975; 55: 383-417
27. Strauss O. The retinal pigment epithelium in visual function. Physiol Rev. 2005; 85:845-881.
28. Tranos PG, Wickremasinghe SS, Stangos NT, Topouzis F, Tsinopoulos I, Pavesio CE. Macular edema. Surv Ophthalmol. 2004; 49:470-490.
29. Rizzolo LJ, Peng S, Luo Y, Xiao W. Integration of tight junctions and claudins with the barrier functions of the retinal pigment epithelium. Prog Retin Eye Res. 2011; 30:296-323.
30. Kaur C, Foulds WS, Ling EA. Blood-retinal barrier in hypoxic ischaemic conditions: basic concepts, clinical features and management. Prog Retin Eye Res. 2008; 27:622-647.
31. Erickson KK, Sundstorm JM, Antonetti DA. Vascular permeability in ocular disease and the role of tight junctions. Angiogenesis. 2007;; 10:103-117.
32. Dardik R, Livnat T, Nisgav Y, Weinberger D. Enhancement of angiogenic potential of endothelial cells by contact with retinal pigment epithelial cells in a model simulating pathological conditions. Invest Ophthalmol Vis Sci. 2010; 51:6188-6195.
33. Stefánsson E, Geirsdóttir A, Sigurdsson H. Metabolic physiology in age related macular degeneration. Prog Retin Eye Res. 2011; 30:72-80.
34. Schulze S, Hoerle S, Mennel S, Kroll P. Vitreomacular traction and exudative age-related macular degeneration. Acta Ophthalmol. 2008; 86:470-481.
35. Inoue Y, Yanagi Y, Matsuura K, Takahashi H, Tamaki Y, Araie M. Expression of hypoxia-inducible factor 1alpha and 2alpha in choroidal neovascular membranes associated with age-related macular degeneration. Br J Ophthalmol. 2007; 91:1720-1721.
36. Grunwald J, Metelitsina T, DuPont J, Ying G-S, Maguire M. Reduced foveolar choroidal blood flow in eyes with increasing AMD severity. Invest Ophthalmol Vis Sci. 2005; 46:1033-1038.
37. Grunwald J, Hariprasad S, DuPont J, et al. Foveal choroidal blood flow in age-related macular degeneration (AMD). Invest Ophthalmol Vis Sci. 1998; 39:385-390.
38. Chen J, Fitzke F, Pauleikhoff D, Bird A. Functional loss in age-related Bruch's membrane change with choroidal perfusion defect. Invest Ophthalmol Vis Sci. 1992; 33:334-340.
39. Friedman E, Krupsky S, Lane A, et al. Ocular blood flow velocity in age-related macular degeneration. Ophthalmology. 1995; 102:640-646.
40. Ciulla T, Harris A, Chung H. Color Doppler imaging discloses reduced ocular blood flow velocities in non-exudative age-related macular degeneration. Am J Ophthalmol. 1999; 128:75-80.
41. Feigl B, Brown B, Lovie-Kitchin J, Swann P. Functional loss in early age-related maculopathy:the ischaemia postreceptoral hypothesis. Eye. 2007; 21:689-696.
42. Witmer A, Vrensen G, Van Noorden C, Schlingemann R. Vascular endothelial growth factors and angiogenesis in eye disease. Prog Ret Eye Res. 2003; 22:1-29.
43. Curcio C. Photoreceptor topography in aging and age-related maculopathy. Eye. 2001; 15:376-383.
44. Sarks J, Sarks S, Killingworth M. Evolution of geographic atrophy of the retinal pigment epithelium. Eye. 1988; 2:552-577.
45. Marmor M, Donovan W, Gaba D. Effects of hypoxia and hyperoxia on the human standing potential. Doc Ophthalmol. 1985; 60:347-352.
46. Tinjust D, Kergoat H, Lovasik J. Neuroretinal function during mild systemic hypoxia. Aviat Space Environ Med. 2002; 73:1189-1194.
47. Kergoat H, Herard M-E, Lemay M. RCG sensitivity to mild systemic hypoxemia. Invest Ophthalmol Vis Sci. 2006; 47:5423-5427.
48. Singh R, Kaiser PK. Advances in AMD imaging. Int Ophthalmol Clin. 2007; 47:65-74.
49. Drexler W, Fujimoto JG. State-of-the-art retinal optical coherence tomography. Prog Retin Eye Res. 2008; 27:45-88.
50. Schmitz-Valckenberg S, Holz FG, Bird AC, Spaide RF. Fundus autofluorescence imaging:review and perspectives. Retina. 2008; 28:385-409.
51. Podoleanu AG, Rosen RB. Combinations of techniques in imaging the retina with high resolution. Prog Retin Eye Res 2008; 27:464-499.
52. Nelson DA, Krupsky S, Pollack A, et al. Special report:noninvasive multiparameter functional optical imaging of the eye. Ophthalmic Surg Lasers Imaging. 2005; 36:57-66.
53. Izhaky D, Nelson DA, Burgansky-Eliash Z, Grinvald A. Functional imaging using the retinal function imager:direct imaging of blood velocity, achieving fluorescein angiography-like images without any contrast agent, qualitative oximetry, and functional metabolic signals. Jpn J Ophthalmol. 2009; 53:345-351.
54. Grinvald A, Bonhoeffer T, Vanzetta I, et al. High-resolution functional optical imaging:from the neocortex to the eye. Ophthalmol Clin North Am. 2004; 17:53-67.
55. Nelson DA, Burgansky-Eliash Z, Barash H, et al. High-resolution wide-field imaging of perfused capillaries without the use of contrast agent. Clin Ophthalmol. 2011; 5:1095-1106.
56. Barak A, Burgansky-Eliash Z, Barash H, Nelson DA, Grinvald A, Loewenstein A. The effect of intravitreal bevacizumab (Avastin) injection on retinal blood flow velocity in patients with choroidal neovascularization. Eur J Ophthalmol. 2011 Nov 7 [Epub ahead of print]
57. Landa G, Garcia PM, Rosen RB. Correlation between retina blood flow velocity assessed by retinal function imager and retina thickness estimated by scanning laser ophthalmoscopy /optical coherence tomography. Ophthalmologica. 2009; 223:155-161.
58. Kwan AS, Barry C, McAllister IL, Constable I. Fluorescein angiography and adverse drug reactions revisited:the Lions Eye experience. Clin Exp Ophthalmol. 2006; 34:33-38.
59. Stefansson E, Machemer R, de Juan E Jr, McCuen BW 2nd, Peterson J. Retinal oxygenation and laser treatment in patients with diabetic retinopathy. Am J Ophthalmol. 1992; 113:36-38.
60. Tiedeman JS, Kirk SE, Srinivas S, Beach JM. Retinal oxygen consumption during hyperglycemia in patients with diabetes without retinopathy. Ophthalmology. 1998; 105:31-36.