PEER REVIEWED
New Horizons in Retinal Laser Treatment
Sublethal retinal laser therapy.
Yannis M. Paulus, MD • Christopher Sramek, PhD • Mark S. Blumenkranz, MD • Daniel Palanker, PhD
Retinal laser photocoagulation was first described 45 years ago and remains the standard of care for many retinal diseases, including diabetic macular edema, proliferative diabetic retinopathy, vascular occlusions, central serous chorioretinopathy (CSC) and retinal tears.1,2 The classic photocoagulation lesion parameters have recently been refined through a series of innovations to minimize the side effects while retaining the therapeutic effect. These innovations include modulations in pulse duration,3,4 lesion intensity5,6 and axial lesion localization.8 In this article, we discuss recent studies demonstrating the motivation, basic science and clinical evidence for the therapeutic role of sublethal retinal laser therapy, and we discuss future directions in sublethal retinal treatment.
THE MOTIVATION FOR SUBLETHAL LASER THERAPY
Laser therapy is typically titrated to a visible clinical effect (graying or whitening of the retina), which corresponds to necrosis of the photoreceptors and, at higher settings, to the inner retina. Although proved effective clinically, this process can lead to unwanted side effects, including post-application lesion enlargement, decreased color and night vision, immediate scotomata8-10 and complications that include choroidal neovascularization,11-12 subretinal fibrosis13,14 and progressive late visual field loss.15-17
One method to provide retinal phototherapy without severe permanent damage is selective treatment of the retinal pigment epithelium by decreasing the laser pulse duration, termed selective retinal therapy (SRT). It has been demonstrated that microsecond (µs) pulse durations can selectively destroy RPE without damaging the retina.18,19 Due to heat confinement, microsecond and nanosecond laser pulses can produce explosive vaporization of melanosomes, resulting in selective damage to the RPE layer, sparing photoreceptors and the inner retina. Subsequent RPE proliferation and migration restores RPE continuity.
The first RPE-selective retinal laser treatment was achieved using 5 µs argon laser pulses at 514 nm and a repetition rate of 500 Hz.20 Currently, several microsecond-pulsed laser systems are available.21,22 Recently, an alternative SRT approach has been developed based on a rapidly scanning continuous wave laser, such that the RPE cells are exposed to laser light for several microseconds.23,24 Several small clinical studies have shown the efficacy of SRT in diabetic maculopathy, CSC25-27 and subfoveal fluid after rhegmatogenous retinal detachment.28
Two other approaches to laser therapy, which are not only subvisible but are actually sublethal, will be the focus of this article. One approach to non-damaging retinal treatment is transpupillary thermotherapy (TTT). In a more intense, destructive modality, it is occasionally used for the treatment of thinner choroidal melanomas.29,30 TTT involves long exposures (~60 s) of a large spot (1.2 to 3 mm) at low irradiance (~10 W/cm2), using a near-infrared (NIR) laser.
In addition to the long exposures of large spots used in TTT, shorter bursts of NIR radiation (810 nm) with small spot sizes (100 to 200 µm) have also been applied for non-damaging retinal phototherapy, termed subvisible diode micropulse (SDM) photocoagulation. In this technique, treatment of retinal vascular disease is performed with the express goal of avoiding any cellular destruction at all. Mathematical models of SDM have demonstrated that the hyperthermia in this approach does not exceed the threshold of cell toxicity and has a high degree of selectivity (Figure 1).31 The low-intensity/high-density SDM allows for complete and confluent (high-density) coverage of the entire diseased retina, such as the areas of macular thickening constituting DME.32-34
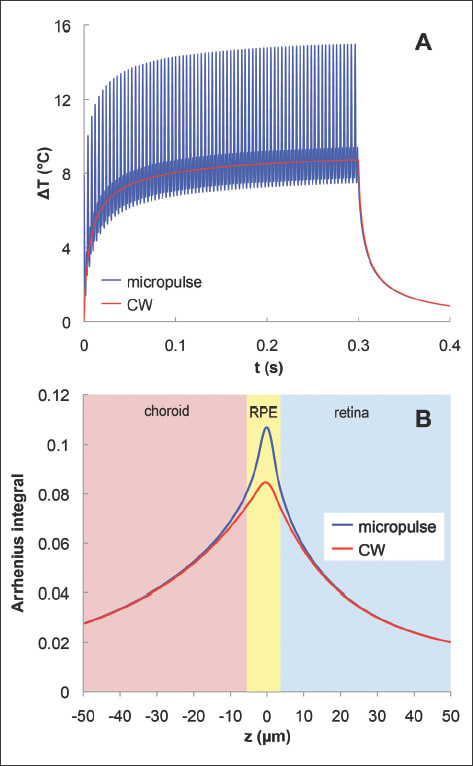
Figure 1. Computational model of the retinal temperature rise and cellular damage produced by continuous and micropulse laser exposure. (A) Temperature rise at the RPE in the center of the laser spot during micropulse (blue, 500 Hz) and continuous wave (red) exposures with 300 ms pulse duration and 14.25 mJ pulse energy. (B) Arrhenius integral, a measure of cellular damage with a threshold of viability equal to 1 in the retina, RPE, and choroid from continuous and micropulse laser systems. This shows the similarity in average temperature and spatial localization of the Arrhenius integral to the RPE and surrounding layers in both laser systems. Reproduced with permission, copyright held by the Association for Research in Vision and Ophthalmology.31
THE BASIC SCIENCE OF SUBLETHAL LASER THERAPY
The exact mechanism by which laser therapy achieves its therapeutic endpoint is an area of active investigation. Classically, it was thought that retinal photocoagulation produced its therapeutic benefit in vascular retinal disease through destruction of retinal cells in the poorly perfused portions of the retina, thus limiting the ischemia and decreasing the production of angiogenic factors. Because photoreceptors are by far the most numerous and most metabolically demanding retinal cells (having many mitochondria), photocoagulation primarily targets the photo-receptor layer and involves the purposeful destruction of a fraction of the photoreceptors to reduce the retinal oxygen demand.
However, recent studies have demonstrated that the science behind photocoagulation is more complicated and that heating produces a constellation of changes at the molecular level in individual cells. Several mechanisms were suggested to underlie the efficacy of panretinal photocoagulation and other laser treatments. These mechanisms include improved retinal oxygenation, reduction in metabolic activity, inhibition of angiogenic stimulators, increased production of angio-inhibitory factors, and oxidative stress.
It has been proposed that laser treatment results in an increase in free-radical activity by direct thermal injury or oxygen reperfusion, and it has been shown that photocoagulation does create a surge in free radicals.35,36 The matrix metalloproteinases (specifically, MMP-9 and alpha2M) have also been noted to increase dramatically in activity after PRP.37 Transforming growth factor-β 2 (TGF-β2) has also been shown to increase dramatically following laser injury.38 Angiostatin and other modulators of vascular endothelial growth factors and fibroblasts have also been noted to modulate the therapeutic effects of PRP and have been implicated in the therapeutic effects of laser.39 Given that the RPE reaches the highest temperature during laser application, it has been postulated that, when RPE is not destroyed by laser or when it is damaged but proliferates and redistributes later to cover the wound, its response to injury is the basis of laser therapy through modulation of local cytokines, including VEGF, pigment epithelial-derived factor, MMPs and tissue inhibitor of matrix metalloproteinases.
Recent experiments looking at rat models of hypoxia have found that photoreceptors act as a repository of growth factors in hypoxic conditions, which cause the angiogenesis and increased vascular permeability characteristic of these conditions.40 This discovery suggests that selective destruction of photoreceptors, while sparing the inner retina, should not only increase availability of oxygen but also reduce the angiogenic and permeability factors.
The pathophysiology underlying a number of retinal disorders has implicated inflammation and hypoxia, inducing angiogenic factors such as VEGF or inflammatory cytokines that stimulate neovascularization or vascular permeability.41-43 Laser photocoagulation is postulated to decrease secretion of angiogenic factors such as VEGF by destroying a fraction of retinal cells. The underlying mechanism of TTT and micropulse treatment is hypothesized to be tissue hyperthermia below the threshold of cell death.
One group of indicators of cellular response to stress that have been actively studied are heat shock proteins (HSPs), which are expressed in response to cellular stress. HSPs assist in the refolding of denatured proteins as chaperone proteins.44 They also inhibit inappropriate protein aggregation, traffic target proteins for degradation or repair, and stabilize the cytoskeleton to maintain cell structure.45 HSPs are expressed at low levels at baseline, but rapidly increase expression in states of thermal, ischemic, and oxidative stress, and they are believed to be a significant component in conferring thermotolerance to heated tissue.46
The HSPs also act on the apoptotic and inflammatory pathways. They interfere with both caspase-dependent and caspase-independent apoptotic cascades in various tissue types, including neural tissue such as retinal ganglion cells.47 HSP70 upregulates the anti-apoptotic protein Bcl-2, and may also prevent mitochondrial cytochrome c release. HSP70, one particular HSP that is 70 kD in size, prevents formation of the complex central to caspase-dependent apoptosis, inhibits caspase-3 activation, and interferes with the caspase-independent apoptosis-inducing factor.48,49 HSP possibly interacts with NFkB, a transcription factor associated with genes involved in inflammation, and its inhibitor IkB to reduce TNF-α.
Expression of HSP has been demonstrated in response to laser treatment in the choroid and retina of rabbit and rodent models.50,51 Using a transgenic mouse having a luminescent indicator (luciferase) of HSP70 expression, it has been found that laser treatment at half the threshold power of RPE damage (at 100 ms, 532 nm, 400 µm diameter) induced transcription of HSP70, an indication of cellular response to sublethal thermal stress (Figure 2).31
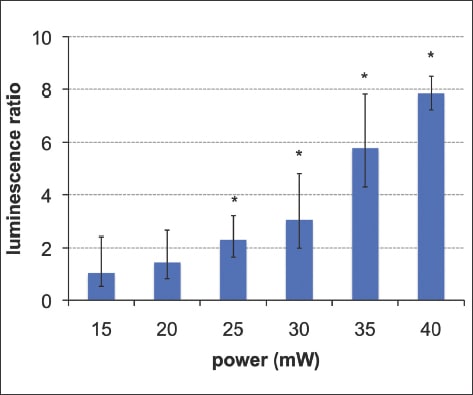
Figure 2. The mean Hsp70 bioluminescence signals from five mice (error bars showing the 95% confidence interval), demonstrating that Hsp70 expression begins to increase at 20 mW, a power half that of visible damage of 40mW, and reaches statistical significance at 25 mW (star indicates p<0.05). Reproduced with permission, copyright held by the Association for Research in Vision and Ophthalmology.31
THE CLINICAL EVIDENCE FOR SUBLETHAL LASER THERAPY
Several trials have demonstrated the clinical efficacy of sublethal phototherapy of the retina in various ocular conditions. Transpupillary thermotherapy has been shown in a retrospective review of 77 patients with occult CNV to result in visual stabilization (47%) or improvement (22%) in the majority of patients.52 Other retrospective reviews have similarly shown a closure of approximately 75% of classic and occult CNV membranes in patients after TTT treatment.53
However, severe concerns have been raised about retinal damage and side effects resulting from TTT therapy,54 including retinal vascular occlusion, retinal neovascularization, retinal traction, vitreous hemorrhage, worsening macular edema, macular infarction, retinal detachment, retinal pigment epithelium tears, and even immediate severe vision loss.
The micropulsed 810 nm diode, low-intensity/high-density subvisible photocoagulater has also been shown to be clinically effective in the treatment of DME and PDR in small clinical series. SDM has been shown to be as effective as modified Early Treatment Diabetic Retinopathy Study laser photocoagulation in the treatment of clinically significant DME without producing visible retinal damage (Figure 3). Beneficial effects in the treatment of neovascularization and macular edema have been recorded in clinical exams and by retinal thickness measurements with optical coherence tomography. These studies, however, are limited by their size and the duration of patient follow-up.31-33
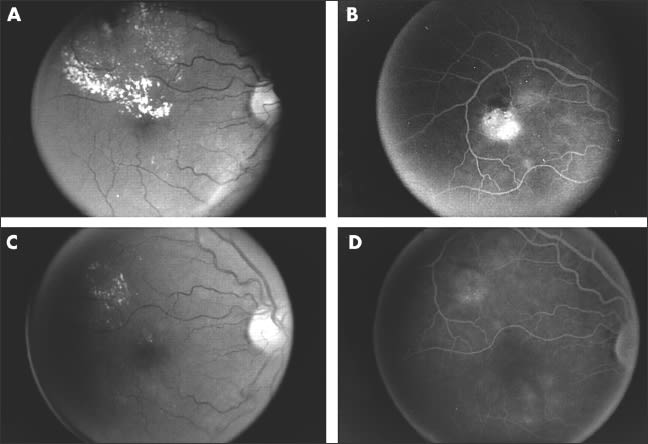
Figure 3: Patient with clinically significant diabetic macular edema (CSME) treated by SDM. (A) Prelaser fundus photograph showing CSME. (B) Prelaser fluorescein angiogram showing focal microvascular leakage. (C) Ten months post-SDM focal treatment fundus photography showing resolution of macular edema and exudates. (D) Ten months post-SDM fluorescein angiogram showing diminished microvascular leakage without scarring. Reproduced with permission from the British Journal of Ophthalmology.32
FUTURE HORIZONS FOR SUBLETHAL LASER THERAPY
Recent advances in laser technology have created new modalities that result in minimal tissue damage while potentially retaining the therapeutic effects of laser therapy for certain clinical conditions. Thermal damage in conventional laser systems limits their utility in treating the macula due to secondary effects, such as scarring. The recently developed improvements minimize or eliminate this side effect.55
Animal studies have indicated that laser power half that of minimally visible burns produces cellular changes while avoiding severe tissue injury.56 This allows it to elicit a biological response without causing permanent cellular death. Further studies are needed to elucidate the mechanisms of cellular response leading to clinical benefits.
To prove the clinical efficacy of these new modalities, larger clinical trials with longer follow-up are required to ensure that these meet or exceed the current clinical standard of care. More trials are also needed of laser treatment in combination therapy with pharmacologic therapy, such as concomitant laser and steroid therapy for retinal vascular occlusions or anti-VEGF therapy.
Continuous innovations in laser technology and progress in understanding of retinal pathology make us believe that improvements in the treatment of retinal diseases using laser therapy will continue for many years to come. RP
REFERENCES
1. Kapany NS, Peppers NA, Zweng HC, Flocks M. Retinal Photocoagulation by Lasers. Nature. 1963;199:146-149.
2. Little HL, Zweng HC, Peabody RR. Argon laser slit-lamp retinal photocoagulation. Trans Am Acad Ophthalmol Otolaryngol. 1970;74:85-97.
3. Blumenkranz MS, Yellachich D, Andersen DE, et al. Semiautomated patterned scanning laser for retinal photocoagulation. Retina. 2006;26:370-376.
4. Jain A, Blumenkranz MS, Paulus Y, et al. Effect of pulse duration on size and character of the lesion in retinal photocoagulation. Arch Ophthalmol. 2008; 126:78-85.
5. Bandello F, Polito A, Del Borrello M, et al. “Light” versus “classic” laser treatment for clinically significant diabetic macular oedema. Br J Ophthalmol. 2005;89:864-870.
6. Bandello F, Brancato R, Menchini U, et al. Light panretinal photocoagulation (LPRP) versus classic panretinal photocoagulation (CPRP) in proliferative diabetic retinopathy. Semin Ophthalmol. 2001;16:12-18.
7. TAP Study Group. Photodynamic therapy of subfoveal choroidal neovascularization in age-related macular degeneration with verteporfin. One-year results of 2 randomised clinical trials — TAP report 1. Arch Ophthalmol. 1999;117:1329-1345.
8. Schatz H, Madeira D, McDonald HR, Johnson RN. Progressive enlargement of laser scars following grid laser photocoagulation for diffuse diabetic macular edema. Arch Ophthalmol. 1991;109:1549-1551.
9. Morgan CM, Schatz H. Atrophic creep of the retinal pigment epithelium after focal macular photocoagulation. Ophthalmology. 1989;96:96-103.
10. Focal photocoagulation treatment of diabetic macular edema. Relationship of treatment effect to fluorescein angiographic and other retinal characteristics at baseline: ETDRS report no. 19. Early Treatment Diabetic Retinopathy Study Research Group. Arch Ophthalmol. 1995;113:1144-1155.
11. Lewen RM. Subretinal neovascularization complicating laser photocoagulation of diabetic maculopathy. Ophthalmic Surg. 1988;19:734-737.
12. Lewis H, Schachat AP, Haimann MH, et al. Choroidal neovascularization after laser photocoagulation for diabetic macular edema. Ophthalmology. 1990; 97:503-510.
13. Guyer DR, D'Amico DJ, Smith CW. Subretinal fibrosis after laser photocoagulation for diabetic macular edema. Am J Ophthalmol. 1992;113:652-656.
14. Rutledge BK, Wallow IH, Poulsen GL. Sub-pigment epithelial membranes after photocoagulation for diabetic macular edema. Arch Ophthalmol. 1993; 111:608-613.
15. Striph GG, Hart WM Jr, Olk RJ. Modified grid laser photocoagulation for diabetic macular edema. The effect on the central visual field. Ophthalmology. 1988;95:1673-1679.
16. Hudson C, Flanagan JG, Turner GS, et al. Influence of laser photocoagulation for clinically significant diabetic macular oedema (DMO) on short-wavelength and conventional automated perimetry. Diabetologia. 1998;41:1283-1292.
17. Ishiko S, Ogasawara H, Yoshida A, Hanada K. The use of scanning laser ophthalmoscope microperimetry to detect visual impairment caused by macular photocoagulation. Ophthalmic Surg Lasers. 1998;29:95-98.
18. Roider J. Laser treatment of retinal diseases by subthreshold laser effects. Semin Ophthalmol. 1999;14:19-26.
19. Brinkmann R, Roider J, Birngruber R. Selective retina therapy (SRT): a review on methods, techniques, preclinical and first clinical results. Bull Soc Belge Ophtalmol. 2006;302:51-69.
20. Roider J, Michaud NA, Flotte TJ, Birngruber R. Response of the retinal pigment epithelium to selective photocoagulation. Arch Ophthalmol. 1992;110:1786-1792.
21. Framme C, Schuele G, Roider J, et al. Threshold determinations for selective retinal pigment epithelium damage with repetitive pulsed microsecond laser systems in rabbits. Ophthalmic Surg Lasers. 2002;33:400-409.
22. Roider J, Brinkmann R, Wirbelauer C, et al. Retinal sparing by selective retinal pigment epithelial photocoagulation. Arch Ophthalmol. 1999;117:1028-1034.
23. Framme C, Alt C, Schnell S, et al. Selective targeting of the retinal pigment epithelium in rabbit eyes with a scanning laser beam. Invest Ophthalmol Vis Sci. 2007;48:1782-1792.
24. Paulus YM, Jain A, Nomoto H, et al. Selective retinal therapy with microsecond exposures using a continuous line scanning laser. Retina. 2010 Oct 6. [Epub ahead of print]
25. Roider J, Brinkmann R, Wirbelauer C, et al. Subthreshold (retinal pigment epithelium) photocoagulation in macular diseases: a pilot study. Br J Ophthalmol. 2000;84:40-47.
26. Elsner H, Porksen E, Klatt C, et al. Selective retina therapy in patients with central serous chrioretinopathy. Graefes Arch Clin Exp Ophthal. 2006;244:1638-1645.
27. Brinkmann R, Roider J, Birngruber R. Selective retina therapy (SRT): a review on methods, techniques, preclinical and first clinical results. Bull Soc Belge Ophtalmol. 2006;302:51-69.
28. Koinzer S, Elsner H, Klatt C, et al. Selective retina therapy (SRT) of chronic subfoveal fluid after surgery of rhegmatogenous retinal detachment: three case reports. Graefes Arch Clin Exp Ophthalmol. 2008;246:1373-1378.
29. Parrozzani R, Boccassini B, De Belvis V, et al. Long-term outcome of transpupillary thermotherapy as primary treatment of selected choroidal melanoma. Acta Ophthalmol. 2009;87:789-792.
30. Aaberg TM Jr, Bergstrom CS, Hickner ZJ, Lynn MJ. Long-term results of primary transpupillary thermal therapy for the treatment of choroidal malignant melanoma. Br J Ophthalmol. 2008;92:741-746.
31. Sramek C, Mackanos M, Spitler R, et al. Non-damaging retinal phototherapy: dynamic range of heat shock protein expression. Invest Ophthalmol Vis Sci. 2010 Nov 18. [Epub ahead of print]
32. Luttrull JK, Musch DC, Mainster MA. Subthreshold diode micropulse photocoagulation for the treatment of clinically significant diabetic macular oedema. Br J Ophthalmol. 2005;89:74-80.
33. Luttrull JK, Musch DC, Spink CA. Subthreshold diode micropulse panretinal photocoagulation for proliferative diabetic retinopathy. Eye (Lond). 2008; 22:607-612.
34. Luttrull JK, Spink CJ. Serial optical coherence tomography of subthreshold diode laser micropulse photocoagulation for diabetic macular edema. Ophthalmic Surg Lasers Imaging. 2006;37:370-377.
35. Jennings PE, MacEwen CJ, Fallon TJ, et al. Oxidative effects of laser photocoagulation. Free Radic Biol Med. 1991;11:327-330.
36. Caldwell RB, Bartoli M, Behzadian MA, et al. Vascular endothelial growth factor and diabetic retinopathy: role of oxidative stress. Curr Drug Targets.2005;6:511-524.
37. Sanchez MC, Luna JD, Barcelona PF, et al. Effect of retinal laser photocoagulation on the activity of metalloproteinases and the alpha(2)-macroglobulin proteolytic state in the vitreous of eyes with proliferative diabetic retinopathy. Exp Eye Res. 2007;85:644-650.
38. Matsumoto M, Yoshimura N, Honda Y. Increased production of transforming growth factor-beta 2 from cultured human retinal pigment epithelial cells by photocoagulation. Invest Ophthalmol Vis Sci. 1994;35:4245-4252.
39. Spranger J, Hammes HP, Preissner KT, et al. Release of the angiogenesis inhibitor angiostatin in patients with proliferative diabetic retinopathy: association with retinal photocoagulation. Diabetologia. 2000;43:1404-1407.
40. Stefansson E, Hatchell DL, Fisher BL, Sutherland FS, Machemer R. Panretinal photocoagulation and retinal oxygenation in normal and diabetic cats. Am J Ophthalmol. 1986;101:657-664.
41. Arjamaa O, Nikinmaa M. Oxygen-dependent diseases in the retina: role of hypoxia-inducible factors. Exp Eye Res. 2006;83:473-483.
42. Arjamaa O, Nikinmaa M, Salminen A, Kaarniranta K. Regulatory role of HIF-1 alpha in the pathogenesis of age-related macular degeneration (AMD). Ageing Res Rev. 2009;8:349-358.
43. Klein R, Klein BE, Knudtson MD, et al. Systemic markers of inflammation, endothelial dysfunction, and age-related maculopathy. Am J Ophthalmol. 2005;140:35-44.
44. Beckham JT, Wilmink GJ, Mackanos MA, et al. Role of HSP70 in cellular thermotolerance. Lasers Surg Med. 2008;40:704-715.
45. Lanneau D, de Thonel A, Maurel S, et al. Apoptosis versus cell differentiation: role of heat shock proteins HSP90, HSP70 and HSP27. Prion. 2007; 1:53-60.
46. Rylander MN, Feng Y, Bass J, Diller KR. Thermally induced injury and heat-shock protein expression in cells and tissues. Ann N Y Acad Sci. 2005;1066:222-242.
47. Franklin TB, Krueger-Naug AM, Clarke DB, et al. The role of heat shock proteins Hsp70 and Hsp27 in cellular protection of the central nervous system. Int J Hyperthermia. 2005;21:379-392.
48. Yenari MA, Liu J, Zheng Z, et al. Antiapoptotic and anti-inflammatory mechanisms of heat-shock protein protection. Ann N Y Acad Sci. 2005;1053:74-83.
49. Awasthi N, Wagner BJ. Upregulation of heat shock protein expression by proteasome inhibition: an antiapoptotic mechanism in the lens. Invest Ophthalmol Vis Sci. 2005;46:2082-2091.
50. Desmettre T, Maurage CA, Mordon S. Heat shock protein hyperexpression on chorioretinal layers after transpupillary thermotherapy. Invest Ophthalmol Vis Sci. 2001;42:2976-2980.
51. Desmettre T, Maurage CA, Mordon S. Transpupillary thermotherapy (TTT) with short duration laser exposures induce heat shock protein (HSP) hyperexpression on choroidoretinal layers. Lasers Surg Med. 2003;33:102-107.
52. Ahuja RM, Benner JD, Schwartz JC, et al. Efficacy of transpupillary thermother-apy (TTT) in the treatment of occult subfoveal choroidal neovascularization in age-related macular degeneration. Semin Ophthalmol. 2001;16:81-85.
53. Newsom RS, McAlister JC, Saeed M, McHugh JD. Transpupillary thermotherapy (TTT) for the treatment of choroidal neovascularisation. Br J Ophthalmol. 2001;85:173-178.
54. Mason JO 3rd, Colagross CC, Feist RM, et al. Risk factors for severe vision loss immediately after transpupillary thermotherapy for occult subfoveal choroidal neovascularization. Ophthalmic Surg Lasers Imaging. 2008;39:460-465.
55. Paulus YM, Jain A, Gariano RF, et al. Healing of retinal photocoagulation lesions. Invest Ophthalmol Vis Sci. 2008;49:5540-5545.
56. Ohkoshi K, Yamaguchi T. Subthreshold micropulse diode laser photocoagulation for diabetic macular edema in Japanese patients. Am J Ophthalmol. 2010;149:133-139.
57. Sramek C, Paulus Y, Nomoto H, Huie P, Brown J, Palanker D. Dynamics of retinal photocoagulation and rupture. J Biomed Opt. 2009;14(3):034007.
Yannis M. Paulus, MD, is an ophthalmology resident at Stanford University Medical Center. Christopher Sramek, PhD, is a postdoctoral fellow at Stanford. Mark S. Blumenkranz, MD, is professor and chairman of the Stanford University Department of Ophthalmology. Daniel Palanker, PhD, is associate professor and director of research in the ophthalmology department at Stanford. Drs. Palanker and Blumenkranz report significant financial interest in OptiMedica Corporation and Topcon Medical Laser Systems. Dr. Paulus and Dr. Sramek report no financial interest in any product mentioned in this article. Dr. Paulus can be reached at ypaulus@stanford.edu. |